Unraveling Double-Stranded DNA: Structure and Significance
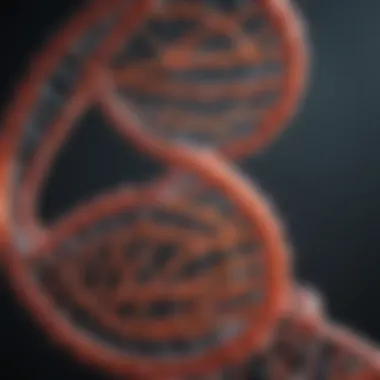
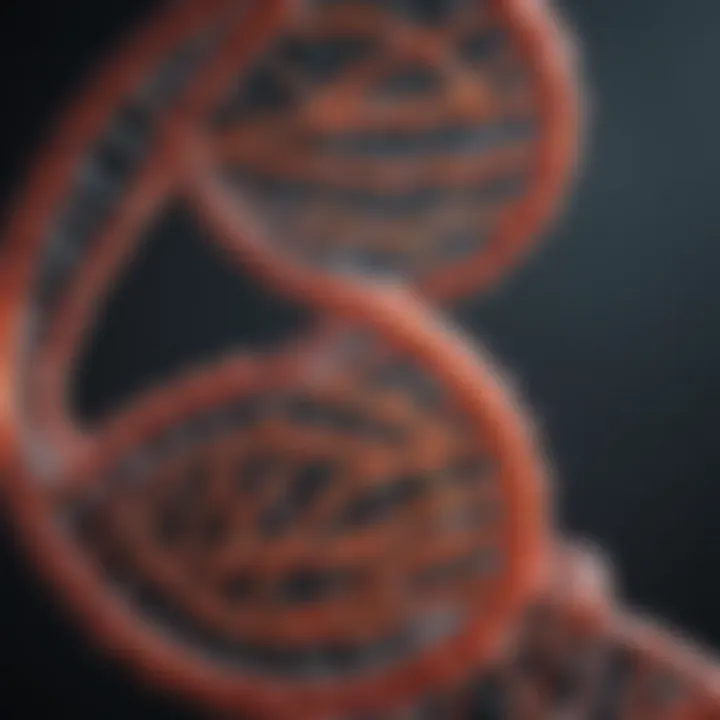
Intro
Double-stranded DNA, or dsDNA, forms the cornerstone of genetic information in living organisms. It is a remarkable structure that not only holds the secrets of life but also exhibits complex properties influencing biological processes. The elegant twist of its double helix shape, discovered by James Watson and Francis Crick, reveals a world of molecular intricacies that drive various functions. Understanding the complexity of dsDNA goes beyond its structural appeal; it encompasses significant implications across multiple scientific fields, including genetics, biotechnology, and medical research.
As we delve into this subject, the interplay between dsDNA's structure and its functional roles will be the focal point. Key findings from recent studies will highlight the importance of the dsDNA arrangement in genetic stability and replication mechanisms. Moreover, the broader implications of dsDNA for genetic engineering and disease research will be considered, showcasing how advancements in molecular biology stem from this fundamental structure. Endeavoring to comprehend these elements can significantly enrich our understanding, particularly for students, researchers, educators, and professionals in the field.
Research Highlights
Key Findings
Recent research has unveiled important findings concerning the structure and function of dsDNA. These findings include:
- Molecular Composition: The basic unit of dsDNA is the nucleotide, each comprising a sugar, a phosphate group, and a nitrogenous base. The sequence and arrangement of these nucleotides encode genetic information.
- Replication Mechanisms: The dynamic process of DNA replication involves unwinding the double helix, where complementary strands serve as templates for new strand formation. This ensures fidelity in genetic inheritance.
- Genetic Stability: The helical structure of dsDNA protects genetic material from damage. This stability is crucial for maintaining genomic integrity over generations.
"Understanding double-stranded DNA provides insight into not only the blueprint of life but also the mechanisms governing cellular functions."
Implications and Applications
The significance of dsDNA extends beyond basic biology. The following are critical areas influenced by its properties:
- Genetics: Advances in genetic engineering rely on knowledge of dsDNA. Techniques like CRISPR utilize this understanding for targeted gene editing, which holds promise for curing genetic disorders.
- Biotechnology: dsDNA plays a vital role in therapeutic developments, including the production of recombinant proteins and the creation of DNA vaccines, which can offer protection against various diseases.
- Disease Research: Studying dsDNA helps elucidate the mechanisms underlying genetic diseases. Insights gained from dsDNA examinations can lead to improved diagnostic tools and treatment strategies.
Methodology Overview
Research Design
To explore the properties of dsDNA, a systematic approach involving both experimental and computational methods is essential. Researchers employ techniques such as X-ray crystallography and Nuclear Magnetic Resonance to elucidate the structural properties of dsDNA.
Experimental Procedures
Key experimental procedures include:
- Sample Preparation: Isolating dsDNA from cellular sources involves various purification techniques, ensuring that the DNA is free from contaminants.
- Structural Analysis: Techniques like X-ray crystallography allow scientists to visualize the molecular arrangements and confirm the helical structure of dsDNA.
- Functional Assays: These involve testing the efficiency of replication and stability under various conditions, providing insights into how dsDNA behaves in different biological contexts.
Understanding the complexities of dsDNA not only enriches the field of molecular biology but also poses essential questions that drive current and future research directions. As the scientific community continues to unravel these complexities, the implications of dsDNA will undoubtedly influence various fields, paving the way for innovations with significant societal impacts.
Foreword to Double-Stranded DNA
The exploration of double-stranded DNA (dsDNA) represents a cornerstone in the field of molecular biology, given its pivotal role in genetic information storage and transmission. Understanding the structure and function of dsDNA is not just an academic endeavor; it has profound implications for how we understand life itself. This section provides a foundational overview crucial for the subsequent discussions on DNA's complexities and significance across various scientific disciplines.
Definition and Basic Structure
Double-stranded DNA is characterized by its helical shape formed by two strands of nucleotides twisted around each other. Each nucleotide consists of three components: a phosphate group, a deoxyribose sugar, and a nitrogenous base. There are four types of nitrogenous bases in DNA: adenine, thymine, cytosine, and guanine. A defining feature of dsDNA is the complementary base pairing: adenine pairs with thymine while guanine pairs with cytosine. This specific pairing not only facilitates the stability of the DNA molecule but also plays a crucial role in replication and transcription processes. The overall structure is often described as a twisted ladder, where the sugar-phosphate backbone forms the sides and paired bases constitute the rungs.
Historical Context of DNA Discovery
The discovery of DNA as the carrier of genetic information unfolded over several key milestones. Initially, Friedrich Miescher isolated nuclein, later known as DNA, from white blood cells in 1869. However, it was not until the 1950s that James Watson and Francis Crick elucidated the double helix structure of DNA, building upon the X-ray diffraction studies of Rosalind Franklin. This groundbreaking revelation linked the molecular structure of DNA to its function in heredity and opened new avenues for genetic research. The combined efforts of these scientists have laid the groundwork for modern genetics, making it possible to understand DNA's role in health, disease, and biotechnological applications.
"The elucidation of the double helix has been described as one of the most significant discoveries in modern biology, fundamentally changing our understanding of genetic inheritance."
In summary, grasping the definition and basic structure of double-stranded DNA, along with its historical context, is essential for comprehending the intricate mechanisms that govern biological processes. It sets the stage for deeper investigations into the chemical composition and functional roles that will be discussed in the following sections.
Chemical Composition of Double-Stranded DNA
The chemical composition of double-stranded DNA is fundamental to understanding its overall structure and function. Each component within the DNA molecule plays a pivotal role in maintaining the integrity and functionality of genetic information. Thus, exploring these components not only highlights their individual significance but also illustrates how they interplay to support life.
Nucleotide Structure
Nucleotides are the building blocks of DNA, and each consists of three components: a phosphate group, a deoxyribose sugar, and a nitrogenous base. The variations in nitrogenous bases—adenine, thymine, guanine, and cytosine—determine the specific information stored within the DNA.
Each nucleotide links together to form a long chain, creating the primary structure of a DNA molecule.
The sequence of these nucleotides encodes genetic information. For instance, the arrangement of bases dictates which proteins a cell produces. Therefore, understanding nucleotide structure is crucial not just for molecular biology, but also for fields like genetics and biotechnology, where manipulating these sequences has significant implications.
Sugar-Phosphate Backbone
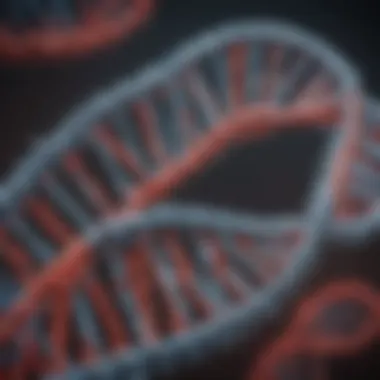
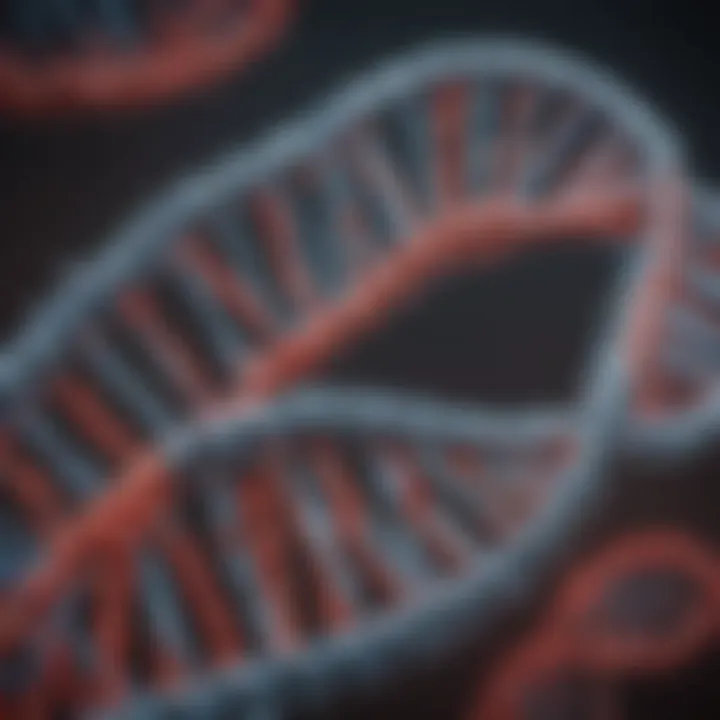
The sugar-phosphate backbone provides structural stability to the DNA molecule. Each sugar molecule in the nucleotide links to a phosphate group from the next nucleotide, creating a repeating pattern along the DNA strand. The sugar is a deoxyribose, which differs from ribose by the absence of one oxygen atom. This minor alteration is crucial because it affects the overall structure and function of nucleic acids.
The backbone serves as a support structure, allowing the bases to project inward, where they can form pairs. This pairing is essential for the double helix formation. The strength and stability of the sugar-phosphate backbone ensure that the genetic information encoded in the base sequences remains intact through cell division and replication.
Base Pairing Mechanisms
Base pairing is one of the most critical aspects of DNA's function. The nitrogenous bases pair according to specific rules: adenine pairs with thymine while guanine pairs with cytosine. These pairs are held together by hydrogen bonds, providing stability while allowing for the necessary unzipping during replication.
Within the double-stranded structure, base pairing provides a mechanism for complementary strands. This ensures accurate replication of genetic material. Errors in base pairing can lead to mutations, which may have various consequences including genetic disorders or increased cancer risk. In biotechnology, understanding these pairing mechanisms is crucial for applications like PCR (Polymerase Chain Reaction) and CRISPR gene editing.
"The precise nature of base pairing is foundational for the fidelity of genetic information transmission."
Three-Dimensional Structure of Double-Stranded DNA
The three-dimensional structure of double-stranded DNA is essential for understanding its function and biological significance. This structure determines how genetic information is stored and accessed in living organisms. The specific arrangements and forms of DNA allow it to effectively participate in replication and transcription processes, ensuring the accurate inheritance of genetic material.
B-Form, A-Form, and Z-Form
Double-stranded DNA can exist in several structural forms, notably the B-form, A-form, and Z-form. The B-form is the most common structure in biological organisms. It is characterized by a right-handed helical shape, with about 10.5 base pairs per turn. This conformation occurs under physiological conditions, allowing optimal interaction with proteins involved in replication and transcription.
The A-form is less common and typically appears in dehydrated environments. It presents a more compact helix with 11 base pairs per turn and is wider than the B-form. This structure may play a role in specific protein-DNA interactions where tighter packing is necessary.
In contrast, the Z-form is a left-handed helix that alternates between purine and pyrimidine bases. It contains 12 base pairs per turn and can form under certain physiological or chemical conditions. Z-form DNA is thought to be involved in gene regulation, indicating that its structure might influence how DNA interacts with other molecules.
Understanding these forms is crucial, as each configuration has distinct properties that influence DNA's biological functions, contributing to areas such as genetic regulation and expression.
Major and Minor Grooves
The three-dimensional structure of DNA also features major and minor grooves which are critical for protein binding. These grooves occur where the two strands of the helix are not tightly bound, allowing access for regulatory proteins and polymerases involved in DNA manipulation.
- Major Groove: The major groove is wider and provides more space for protein recognition. Many transcription factors and regulatory proteins interact here due to this space, allowing them to identify specific sequences of nucleotides. This interaction is key for gene expression modulation.
- Minor Groove: The minor groove, being narrower than the major, has a different profile of interaction. Proteins that bind here often have alternative mechanisms for recognizing DNA sequences. Though less common, it serves important functions in various cellular processes, including DNA repair and replication.
Collectively, the orientation and availability of major and minor grooves in the DNA structure enable a variety of molecular interactions, facilitating essential biological processes like transcription, replication, and repair. Understanding the three-dimensional arrangement provides insights into the mechanics of genetic information flow and regulation.
Mechanisms of DNA Replication
DNA replication is a fundamental process that ensures the accurate copying of genetic material in all living organisms. The significance of this topic lies in its pivotal role in cellular reproduction and the transmission of genetic information across generations. Understanding these mechanisms provides insight into not only how genetic information is preserved but also the implications these processes have on heredity, evolution, and disease.
The replication of DNA is a highly coordinated and complex event. Enzymes play crucial roles in this process, guiding how the double-stranded structure unwinds and how new strands are synthesized. Additionally, the understanding of leading and lagging strand synthesis is essential to comprehend the challenges inherent in duplicating the antiparallel nature of double-stranded DNA. Each element in this process bears substantial importance, influencing both the fidelity of replication and the overall efficiency of cellular operations.
Enzymatic Roles in Replication
Various enzymes are responsible for the replication of DNA, each performing specific functions vital for the accurate duplication process. One of the most well-known enzymes is DNA polymerase. This enzyme is responsible for adding nucleotides to the growing DNA strand complementary to the template strand. In eukaryotes, multiple types of DNA polymerases exist, each with distinct roles in both the leading and lagging strand synthesis.
Another essential enzyme is helicase, which unwinds the double-stranded DNA at the replication fork, separating the two strands to allow access for replication machinery. Along with helicase, single-strand binding proteins (SSBPs) stabilize the separated strands, preventing them from re-annealing prematurely.
Furthermore, primase synthesizes short RNA primers, providing an initiation point for DNA polymerase, which cannot start new strands without a primer.
In summary, the orchestration of these enzymes ensures that replication occurs accurately and efficiently. Without their specific functions, errors in the genetic code could become prevalent, leading to potential mutations.
Leading and Lagging Strand Synthesis
The architecture of double-stranded DNA presents a challenge during replication. DNA strands are antiparallel; consequently, replication must occur differently for the leading and lagging strands. The leading strand is synthesized continuously in the direction of the replication fork, facilitating an unbroken strand of new DNA. In contrast, the lagging strand is synthesized discontinuously, resulting in the formation of short Okazaki fragments. This occurs because polymerase can only add nucleotides in the 5’ to 3’ direction.
The synthesis of the lagging strand involves several steps:
- Forms RNA primers: Primase lays down RNA primers on the lagging strand to initiate replication.
- Synthesis of Okazaki fragments: DNA polymerase adds nucleotides, creating short segments.
- Removal of RNA primers: After synthesis, RNA primers are replaced with DNA nucleotides.
- Ligation of fragments: Finally, DNA ligase connects the Okazaki fragments, creating a continuous strand.
Understanding the complex interactions and processes of DNA replication is crucial in numerous scientific disciplines, from molecular biology to genetics. Issues within these mechanisms can lead to serious consequences, such as genetic disorders and cancer, underlining the importance of careful study in this area.
"The precision of DNA replication is fundamental to maintaining genetic integrity across generations."
Functional Roles of Double-Stranded DNA
Double-stranded DNA (dsDNA) serves two primary functional roles in biology: the storage of genetic information and the regulation of gene expression. Both functions are fundamental for the maintenance and expression of life. Understanding these roles is crucial, as they underscore how genetic information is transmitted and utilized within organisms, affecting everything from cellular functions to the development of disease.
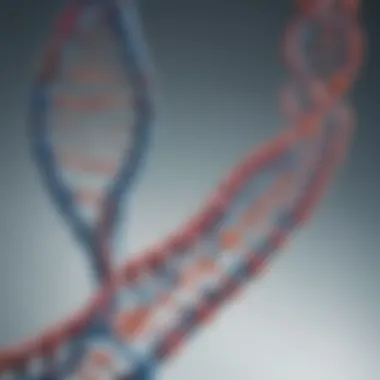
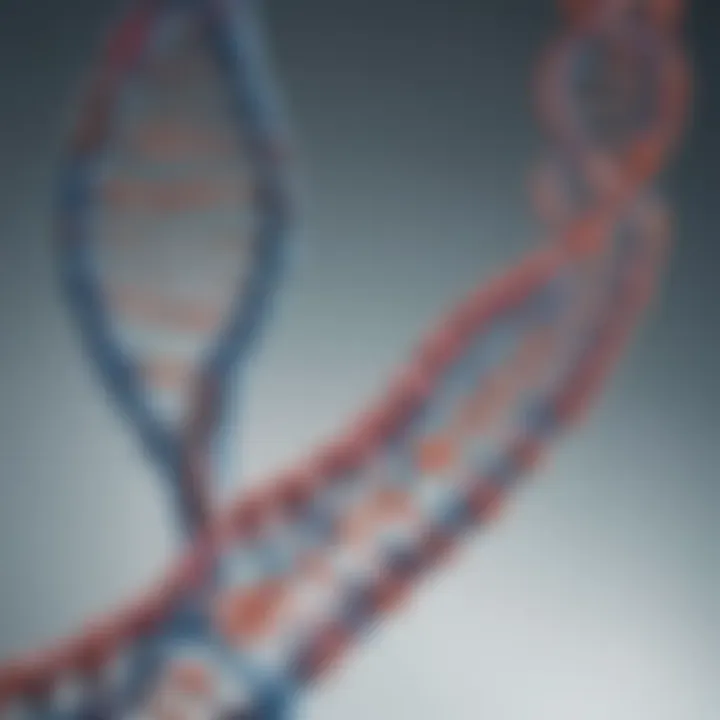
Genetic Information Storage
At its core, dsDNA functions as the repository of genetic information. Each sequence of nucleotides within dsDNA encodes essential instructions required for the synthesis of proteins, which perform a plethora of functions within the cell. The sequences are organized in a manner that allows specific genes to be accessed when needed.
The compact structure of dsDNA allows it to store vast amounts of information within a relatively small space. This efficiency is essential for cellular activities. The specific pairing of bases—cytosine with guanine and adenine with thymine—ensures the fidelity of this information. Any alterations to these sequences can lead to mutations, affecting how proteins are made and potentially leading to diseases.
"The sequence of bases in DNA is a unique code that determines not just the traits of an organism, but also its functioning at the cellular level."
Gene Expression Regulation
In addition to coding for proteins, dsDNA plays a vital role in the regulation of gene expression. Different genes can be turned on or off in response to various internal and external signals. This regulation allows for cellular differentiation and the ability for cells to respond adaptively to their environment.
Several mechanisms facilitate this regulation. For instance, transcription factors are proteins that bind to specific DNA sequences, influencing the transcription of particular genes. Additionally, epigenetic modifications can change how tightly DNA is wound around histones, affecting accessibility for transcription. These modifications may not change the DNA sequence but can drastically influence gene expression.
In summary, the functional roles of double-stranded DNA extend far beyond mere information storage. They encompass intricate processes that determine how, when, and where a particular genetic code is expressed within an organism, making it a cornerstone of molecular biology.
Double-Stranded DNA in Biotechnology
Double-stranded DNA plays a crucial role in biotechnology, influencing methods that transform scientific possibilities into practical applications. The utility of dsDNA in various biotechnological processes highlights its significance across diverse fields. From genetic engineering to synthetic biology, the manipulation and understanding of dsDNA have created remarkable advancements, driving innovation and exploration in molecular biology.
Recombinant DNA Technology
Recombinant DNA technology has revolutionized genetic manipulation. This process involves combining DNA from different sources, creating new genetic combinations that do not occur naturally. Here are some key points on its importance:
- Gene Cloning: Recombinant DNA allows for the cloning of specific genes. By inserting a gene of interest into a vector, researchers can produce large quantities of the gene's product.
- Production of Proteins: This technology enables the production of essential proteins, such as insulin, in microorganisms like E. coli. Such advancements have profound implications in medicine and pharmaceuticals.
- Transgenic Organisms: Recombinant DNA is instrumental in creating transgenic plants and animals. These organisms possess traits from other species, enhancing agricultural productivity, disease resistance, and nutritional value.
Through the power of recombinant DNA technology, researchers can explore new frontiers in biology, addressing problems with innovative solutions.
Despite its benefits, recombinant DNA technology raises ethical concerns. Issues regarding genetic privacy, ecological effects, and potential misuse remain topics of debate among scientists and bioethicists alike.
Gene Editing Techniques
Gene editing techniques have emerged as powerful tools for precise modifications of dsDNA. Such techniques include CRISPR-Cas9, TALENs, and ZFNs, each with distinct characteristics and applications. Consider the following aspects:
- Precision and Efficiency: Technologies like CRISPR-Cas9 enable precise targeting of specific DNA sequences, facilitating accurate gene modifications. This precision minimizes off-target effects, making gene editing more reliable.
- Therapeutic Applications: Gene editing has opened pathways for treating genetic disorders. By correcting mutations at the DNA level, these techniques promise potential cures for diseases previously deemed untreatable.
- Agricultural Improvement: Gene editing can enhance crop traits, such as yield and resistance to pests. This could lead to improved food security in a world facing challenges from climate change.
Gene editing carries ethical considerations, particularly concerning the implications of altering genomes. Questions arise about the long-term effects on ecosystems, species integrity, and potential unintended consequences.
In summary, the integration of double-stranded DNA in biotechnology underpins significant advancements in various industries. Both recombinant DNA technology and gene editing techniques demonstrate the transformative potential of manipulating dsDNA. However, as we progress, balancing innovation with ethical considerations will be essential.
Double-Stranded DNA and Disease Research
Double-stranded DNA (dsDNA) plays a crucial role in disease research. Its structure and stability govern not only genetic inheritance but also the mechanisms behind various diseases. Understanding dsDNA's contributions to health and disease can lead to better diagnostic tools and treatment approaches.
Genetic Disorders and Mutations
Genetic disorders arise from alterations in the DNA sequence. These mutations can be small, such as single nucleotide polymorphisms, or large, involving significant sections of the genome. Analyzing dsDNA enables scientists to identify these mutations and their effects on gene function. For instance, diseases like cystic fibrosis and sickle cell anemia stem from specific mutations in particular genes.
- Types of mutations include:
- Point mutations: change a single nucleotide.
- Insertions/deletions: add or remove nucleotides.
- Copy number variations: involve duplications or deletions of sections of DNA.
The discovery of techniques such as whole-genome sequencing has revolutionized our understanding of genetic disorders. Researchers can now map mutations to their phenotypic consequences. This connection is vital for developing therapies tailored to individual genetic profiles. Additionally, genetic counseling becomes feasible as dsDNA analysis provides insights into inheritance patterns.
Role in Cancer Biology
Cancer biology heavily relies on understanding dsDNA's structure and function. Abnormalities in DNA can lead to uncontrolled cell division and cancer development. Specific mutations within key genes, often referred to as oncogenes and tumor suppressor genes, can initiate and promote cancer progression. For example, mutations in the TP53 gene are observed in various cancers.
Key points in cancer research involving dsDNA include:
- Oncogenes: activate cell growth and division when mutated.
- Tumor suppressor genes: normally inhibit cell division; mutations lead to loss of this control.
Moreover, dsDNA's role in epigenetics, where chemical modifications affect gene expression without altering the underlying sequence, presents new avenues for research. Epigenetic changes can influence the progression of cancer and may serve as targets for novel therapies. Understanding how environmental factors interact with dsDNA further enriches the field of cancer biology.
"The interplay between genetics and environmental factors in manipulating dsDNA holds significant potential for advancements in cancer treatment strategies."
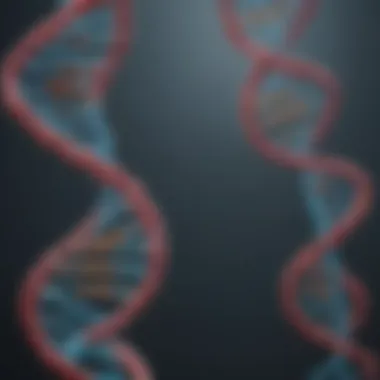
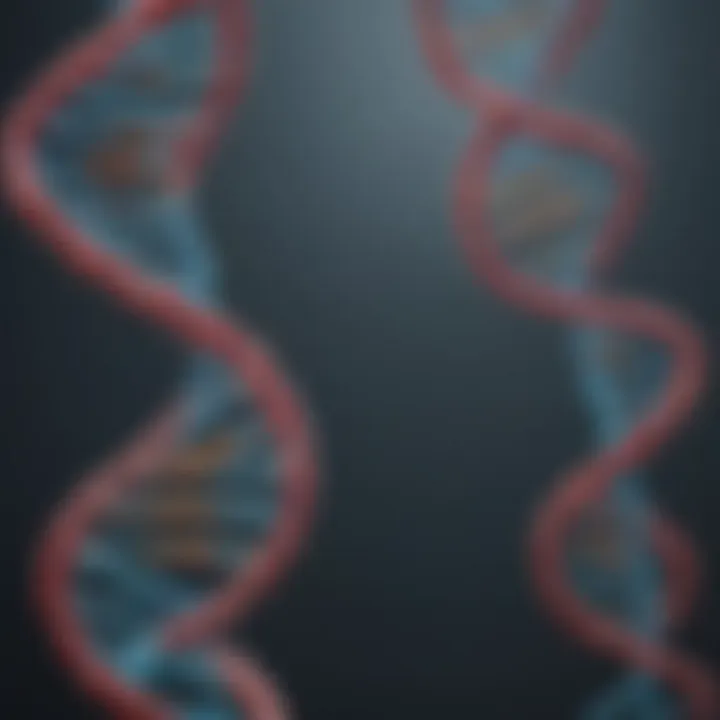
In summary, the study of dsDNA in the context of disease research not only illuminates the pathophysiology of genetic disorders and cancer but also enhances therapeutic strategies. The insights gained from dsDNA analysis promise to improve patient care through more accurate diagnostics and personalized medicine.
Current Trends in DNA Research
Research in DNA has become a focal point in molecular biology. This trend is not just academic; it carries substantial implications for medicine, environmental science, and technology. Keeping pace with advancements is necessary for ensuring that research is relevant and impactful. Key areas showing growth include sequencing technologies and synthetic biology applications.
Advancements in Sequencing Technologies
The field of DNA sequencing has experienced rapid progress over the past few years. Traditional Sanger sequencing has largely been supplemented by next-generation sequencing (NGS) methods. Technologies from Illumina, Pacific Biosciences, and Oxford Nanopore have vastly increased throughput while drastically reducing costs. This democratization of sequencing allows more researchers access to genomic data, facilitating large-scale studies in genetics and oncology.
Benefits of advancements in sequencing technologies include:
- Speed: NGS can process millions of DNA fragments simultaneously, significantly shortening the time needed for comprehensive genomic analysis.
- Accuracy: Improvements continue to lessen error rates associated with sequencing, enhancing the reliability of results.
- Cost-Effectiveness: With lower costs, sequencing can be incorporated into more research projects, from basic science to clinical applications.
These changes also warrant careful consideration regarding data management and bioinformatics. The volume of information generated requires robust systems for storage and analysis, including machine learning methods for interpretation.
"Next-generation sequencing technologies are not just transforming our approach to research but altering our understanding of genetics itself."
Synthetic Biology Applications
Synthetic biology, an interdisciplinary branch combining biology and engineering, is making waves in current DNA research. Using detailed knowledge of the DNA structure allows researchers to design and construct new biological parts, devices, and systems. Applications range from medicine to agriculture and energy.
Developments in synthetic biology include:
- Gene Synthesis: With tools like the GeneART platform, scientists can create custom genes tailored for specific purposes, whether therapeutic or experimental.
- CRISPR Technology: This gene-editing tool has opened possibilities for precise genome modifications. It can target multiple genes across various organisms, thus enhancing research efficiency.
- Biofuel Production: Utilizing engineered organisms for biofuel production presents a promising avenue for renewable energy. This includes the creation of genetically modified bacteria that can produce fuels from non-food sources.
The implications of synthetic biology are immense, but ethical considerations must not be overlooked. Issues of biosafety, ethical use, and potential ecological effects need careful evaluation to guide responsible research practices.
Ethical Considerations in DNA Research
The rapid advancements in DNA research present not just a frontier of biological discovery, but also a thorough consideration of the ethical landscapes they inhabit. As the tools for manipulating genetic material become more sophisticated, the ethical implications of these procedures demand focus. The exploration of gene therapy, biobanking, and the broader ramifications of DNA technologies raise significant questions. These are not merely academic; they impact societal values, individual rights, and the fabric of human genetics.
Understanding the ethical considerations in this context is crucial. It not only shapes research methodologies but also guides regulatory frameworks designed to protect both current and future generations.
Gene Therapy Ethics
Gene therapy stands as a profound application of DNA research, aiming to correct genetic defects or prevent diseases by modifying genes. However, it entails numerous ethical concerns that professionals must navigate carefully. Among these, the potential for unintended genetic consequences is a primary worry. Adjusting an individual's genetic makeup could have effects that extend beyond the targeted genes, possibly introducing new mutations or unpredicted traits.
Such risks advocate for the thorough review of the patients’ informed consent. This process should involve comprehensive discussions about the prospective benefits and any unknown risks involved. The idea of editing the germline, which affects not only the individual but their offspring, further complicates ethical discussions.
Additionally, there’s a concern regarding accessibility and equity. If gene therapies advance significantly but remain costly, issues of justice arise. Will only affluent individuals gain access to these life-altering treatments? This consideration raises alarms about further widening socio-economic divides, leading to questions surrounding societal responsibility in ensuring equitable health opportunities.
Biobanking and Consent Issues
Biobanking involves storing biological samples for future research and often includes DNA samples. It raises unique ethical considerations chiefly related to consent and the use of biological materials. Individuals providing samples should do so with a clear understanding of how their genetic material may be used in the future. It is crucial that biobanks establish transparent consent processes that offer individuals detailed information about the potential research applications.
More troubling are the instances where samples are used beyond the originally stated purposes. This scenario can lead to ethical misconduct, particularly if donors’ rights are not sufficiently protected.
Furthermore, data privacy poses another significant issue. The possibility of re-identifying individuals from stored genetic data raises worries about confidentiality. Researchers must be diligent in maintaining the anonymity of donor samples to respect privacy rights. Institutions must invest in robust frameworks to secure data while still facilitating scientific exploration.
Epilogue and Future Directions
The exploration of double-stranded DNA (dsDNA) offers vital insights into the foundational principles of molecular biology. This article reveals the complexity and elegance of dsDNA, encompassing its structural features and functional roles across various scientific domains. Recognizing the significance of dsDNA promotes understanding of genetic information mechanisms and biotechnology developments.
Furthermore, as research progresses, the ethical implications of manipulating dsDNA must be prioritized. Knowledge from genetics has the potential to affect health decisions and societal norms profoundly. Thus, future studies should also emphasize responsible practices alongside scientific discovery.
Although established concepts surrounding dsDNA are invaluable, a deeper inquiry into less understood areas remains critical.
"Advancements in dsDNA research open pathways for innovations that can redefine our understanding of genetics and its applications."
Summary of Key Findings
This article highlights several key aspects of double-stranded DNA. First, understanding its chemical composition and unique structural forms allows researchers to appreciate its complexity. Each component, from nucleotides to grooves, plays a crucial role in how genetic information is preserved and utilized.
Moreover, the mechanisms underpinning DNA replication are essential for maintaining genetic fidelity. Functional roles of dsDNA in gene expression and regulation demonstrate its importance in biology. Additionally, dsDNA has profound implications in fields such as biotechnology and disease research, where insights gained can lead to therapeutic advancements.
Potential Areas for Future Research
Numerous avenues warrant exploration in the realm of dsDNA research. Some potential areas include:
- Investigating the structural variations under different environmental conditions to understand stability and functionality.
- Enhancing sequencing technologies to improve accuracy and reduce costs for broader genetic studies.
- Exploring synthetic biology applications to create novel organisms for sustainable solutions.
- Assessing the long-term impacts of gene therapy approaches, focusing on both effectiveness and ethical considerations.
- Examining interactions between dsDNA and various cellular components to decipher gene regulation mechanisms more thoroughly.