Exploring the Multifaceted Functions of RNA
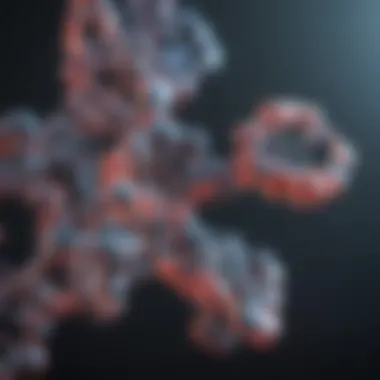
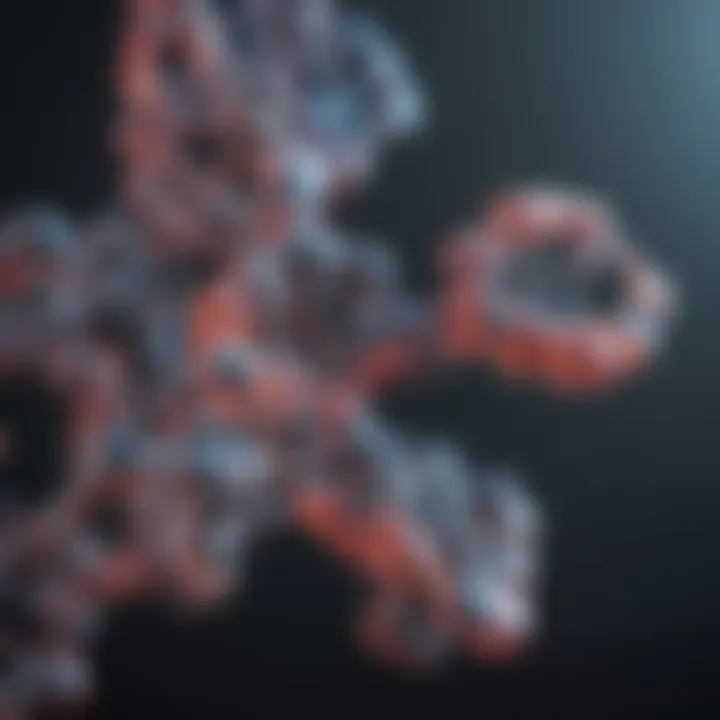
Intro
Ribonucleic acid, better known as RNA, has become a hot topic in the realms of molecular biology and medicine. While many people may think of DNA when it comes to genetic material, RNA is equally significant, playing a variety of roles in cellular function and gene regulation. It isnāt just an intermediary; it actively participates in processes that are fundamental to life.
From serving as a messenger that carries genetic information from DNA to the ribosomes, where proteins are synthesized, to acting as a regulator in complex cellular processes, RNAās versatility cannot be overstated. In recent years, scientific advancements have illuminated not only its traditional roles but also its potential in groundbreaking medical applications. This article will embark on a thorough examination of RNA's functions, covering its structures, types, and the intricate mechanisms of its synthesis. We will also pull back the curtain on how RNA interacts with DNA and proteins, effectively showcasing its indispensable contributions to protein synthesis and gene regulation.
With this exploration, we aim to unearth RNA's complexities and examine its relevance in contemporary biotechnology, providing insights and understanding for an audience that includes students, researchers, educators, and professionals alike. Let's dive in.
Research Highlights
Key Findings
The study of RNA presents multiple intriguing findings:
- Diverse Functions: RNA is not a monolithic entity. Various types exist, including mRNA (messenger RNA), tRNA (transfer RNA), and rRNA (ribosomal RNA), each playing distinct roles in cellular activities.
- Gene Regulation: Small RNA molecules, such as microRNAs and siRNAs, have been found to play critical roles in regulating gene expression. This regulation is crucial in many biological processes and diseases.
- Therapeutic Applications: The use of RNA in therapeutic interventions, like RNA-based vaccines that gained prominence during the COVID-19 pandemic, has opened new avenues in medicine.
Implications and Applications
Understanding RNA is not just an academic endeavor; it has real-world implications:
- Biotechnology: RNA manipulation technologies, such as CRISPR, have revolutionized genetic engineering and offer potential solutions to complex issues like genetic disorders.
- Healthcare: RNAās role in disease mechanisms, especially viral infections, positions it as a target for new drugs and therapeutic strategies.
- Environmental Science: Studying RNA from diverse organisms can provide insights into ecological systems and inform conservation efforts.
"RNA is a chameleon of the molecular world, adapting and modifying its roles as science continues to discover its hidden capabilities."
Methodology Overview
Research Design
To comprehensively explore the functions of RNA, a multidimensional research design is employed, combining theoretical analysis with empirical studies. This approach allows for a richer understanding of RNA's roles within biological systems.
Experimental Procedures
Key experimental procedures in RNA research include:
- RNA isolation: Techniques like extraction with phenol-chloroform are commonly used to isolate RNA from cells.
- Sequencing: Next-generation sequencing methods allow researchers to analyze RNA molecules, revealing information about gene expression and regulation.
- Functional assays: Various assays, such as reporter assays, are undertaken to assess gene regulation mechanisms involving RNA.
Through these methodologies, the intricate tapestry of RNA's functions is unraveled, paving the way for advancements in various fields, from medicine to biotechnology.
Prolusion to Ribonucleic Acid
Ribonucleic Acid, or RNA, is fundamentally woven into the fabric of life. Understanding RNA is like unlocking a treasure chest of biological secrets. This section serves as the gateway, offering insights into what RNA is and why it matters. If we dig deeper, we can appreciate that RNA is not just a player in the game; it often holds the reins, steering processes that are crucial for cellular function.
The significance of RNA extends beyond its molecular structure. It acts as a messenger, transmitting vital genetic information from DNA to the sites of protein synthesis. Moreover, RNA is involved in the regulation of gene expressionāa key mechanism that determines how genes are turned on or off. By grasping the basics of RNA, one sets the stage for exploring its myriad functions and implications in modern science.
Defining RNA
Letās start at the beginning: what exactly is RNA? Ribonucleic Acid is a complex molecule composed of nucleotides, which are the building blocks of genetic material. Each nucleotide consists of three components: a ribose sugar, a phosphate group, and a nitrogenous base. There are four types of nitrogenous bases in RNAāadenine, guanine, cytosine, and uracilāeach playing a unique role in the coding and regulation of genetic information.
RNA operates primarily in single strands, unlike its closely related counterpart, DNA, which forms a double helix. This structural difference is significant; it allows RNA to take on various shapes and functions. For instance, messenger RNA (mRNA) carries the code from DNA to ribosomes, while transfer RNA (tRNA) helps translate that code into proteins. Thus, RNA is not merely a passive messenger; it actively participates in the intricate dance of life at the molecular level.
Historical Context
The story of RNA stretches back over a century, marked by curiosity and scientific breakthroughs. In the early 20th century, scientists were beginning to peel back the layers of DNA and RNA, but it wasn't until the 1950s and 1960s that the significance of RNA truly began to emerge. Researchers like Francis Crick and James Watson laid the groundwork for our understanding of genetic coding, but RNAās essential role was often overshadowed by the focus on DNA.
Notably, the discovery of the role of mRNA in protein synthesis was a pivotal moment in molecular biology. Early experiments by scientists such as Sydney Brenner showcased how RNA plays a critical role in the central dogma of molecular biology: DNA transcribing to RNA, which then translates to proteins.
The importance of these discoveries has rippled through time, shaping our understanding of biology, genetics, and, more recently, biotechnology. Today, as we uncover the complexities of RNAāfrom its types and structures to its functionsāwe stand at the forefront of a new era of scientific exploration. Understanding RNA summarizes the shifts in research paradigms and beckons us to probe deeper into its functions and potentials in health and disease.
The Structure of RNA
Understanding the structure of ribonucleic acid (RNA) is fundamental to grasping its functions within biological systems. The intricacies of RNA's structure influence everything from its role in protein synthesis to its capabilities in gene regulation. Each layer of structure helps define how RNA operates and interacts with various molecular partners. As such, it becomes crucial to dissect the components that contribute to its overall functionality.
Chemical Composition
At its core, RNA is composed of long chains of nucleotides, which act as the building blocks of this vital molecule. Each nucleotide consists of three essential parts: a ribose sugar, a phosphate group, and a nitrogenous base. The ribose sugar distinguishes RNA from DNA, which has deoxyribose. This subtle difference in sugar contributes to RNA's stability and reactivity.
The nitrogenous bases in RNA form the crux of its' potential. They come in four varietiesāadenine, uracil, cytosine, and guanine. Uracil is a distinguishing feature, as it replaces thymine found in DNA. This key variance highlights both functional and structural differences between RNA and DNA, making it capable of forming less stable yet more adaptable structures.
While each of these components is fairly simple on its own, together they create a molecule of remarkable complexity and versatility.
RNA vs DNA
When juxtaposed with DNA, RNA reveals a number of critical distinctions. Firstly, DNA is typically double-stranded and helical, while RNA is predominantly single-stranded, enabling it to fold into complex shapes. This single strand allows RNA to perform a diverse range of functions compared to its more rigid counterpart.
Another noteworthy difference lies in the length and durability of these molecules. DNA, being a stable repository of genetic information, exists in longer and more structured forms, while RNA is generally shorter and often has a transitory lifecycle.
Furthermore, RNAās configurational freedom lets it interact with proteins and ribosomes effectively, enhancing its role in translating genetic information into functional products. In other words:
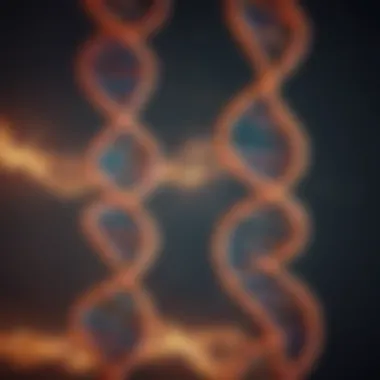
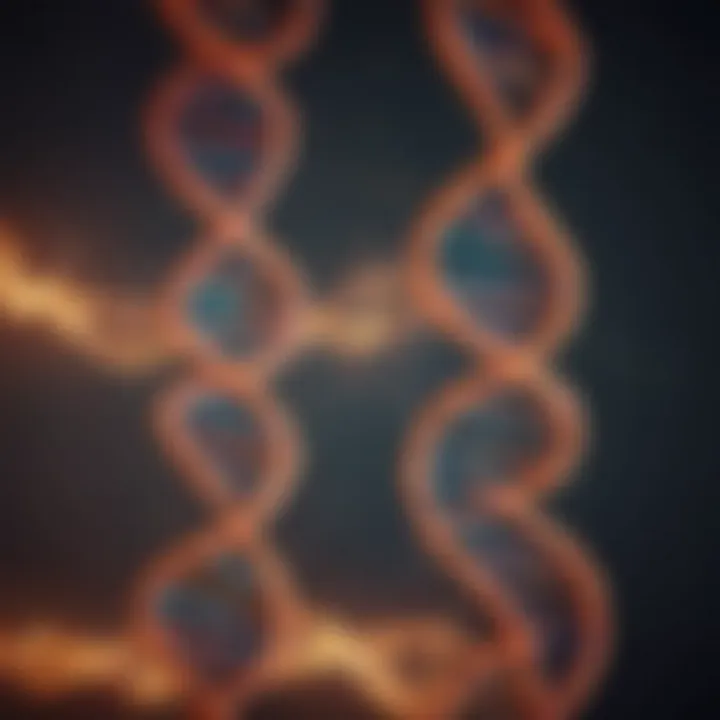
- DNA: Double-stranded, stable, long-lived, primarily stores genetic information.
- RNA: Single-stranded, versatile, shorter-lived, primarily involved in protein synthesis and gene regulation.
Secondary and Tertiary Structures
The structural magic of RNA comes into play primarily in its secondary and tertiary forms. Secondary structures are typically formed through base pairing within the single strand itself, resulting in arrangements such as loops, hairpins, and bulges. These formations are essential for the stability of RNA molecules and also define their functional roles in various biological processes.
Moving further into tertiary structures, the folds and twists become even more pronounced. These configurations allow RNA to engage with proteins and other nucleic acids in very specific ways. Enzymatic RNA, or ribozymes, are especially illustrative of how tertiary structure affects function, enabling RNA to catalyze reactions independently of protein enzymes.
The ability of RNA to adopt multiple structural forms is what gives it a certain dynamism, making it a key player in molecular biology. This flexibility has implications in the broader spectrum of cellular processes, including:
- Gene expression regulation
- Protein synthesis via tRNA and rRNA
- Serving as genetic material in some viruses
"The structural complexity of RNA is paralleled only by its evolutionary significanceāa molecule that bridges the realms of genetics and proteins."
In summary, the structure of RNA is not just a matter of arrangement but fundamentally dictates its varied roles within cells. From its chemical makeup to the delicate dance of secondary and tertiary formations, each aspect is instrumental in enabling RNA to perform its myriad functions.
Types of RNA and Their Functions
Understanding the various types of RNA is crucial in grasping their multifaceted roles within cells. Each type has a unique function that contributes to the overarching process of gene expression and protein synthesis. Their interplay facilitates the transformation of genetic information into functional proteins, which are essential in nearly all cellular activities. In this section, we will explore the major types of RNA: messenger RNA (mRNA), ribosomal RNA (rRNA), transfer RNA (tRNA), and regulatory RNA, highlighting their significance in the realm of molecular biology.
Messenger RNA (mRNA)
Messenger RNA serves as the vital intermediary between DNA and protein synthesis. When a gene is activated, the corresponding DNA sequence is transcribed into mRNA. This process occurs within the nucleus, where the genetic blueprint is copied. The resulting mRNA molecule then transports the genetic instructions, like a delivery driver with a critical package, out into the cytoplasm where ribosomes beckon.
The key functions of mRNA can be summarized as follows:
- Template for Translation: mRNA carries the specific sequences of nucleotides that dictate the order of amino acids in a protein, organized in sets of three known as codons.
- Diverse Forms: mRNA can exist in various forms based on the gene it originates from, impacting how proteins are produced during different cellular conditions.
- Role in Regulating Protein Synthesis: The lifespan of mRNA in the cytosol can affect protein production levels, with some mRNAs being quickly degraded while others are stable and long-lasting.
This direct line of communication between DNA and the cellular machinery is instrumental, devoid of which the cell's protein synthesis machinery could grind to a halt.
Ribosomal RNA (rRNA)
Ribosomal RNA forms the core of ribosome structure and function. These molecules are not merely passive components; they actively engage in decoding mRNA during the translation phase of protein synthesis. The ribosome, which is a complex of rRNA and proteins, is where mRNA and tRNAs converge to facilitate the assembly of amino acids into a polypeptide chain.
Key points about rRNA include:
- Structural Backbone: rRNA makes up the large and small subunits of the ribosome, showcasing an extraordinary ability to catalyze peptide bond formation essential for proteins.
- Facilitation of tRNA Interaction: rRNA plays a crucial role in correctly pairing codons from mRNA with the appropriate tRNA anticodon, ensuring the integrity of translation.
- Ancient Molecular Machinery: rRNA is one of the most conserved sequences in biology, suggesting its fundamental importance across diverse life forms.
This underscores the idea that without rRNA, the ribosomes could not perform their vital role, and protein synthesis would face serious consequences.
Transfer RNA (tRNA)
Transfer RNA acts as the interpreter during protein synthesis, linking the genetic code carried by mRNA to the appropriate amino acids. Picture it as a skilled translator that takes information from one language and converts it into another. Each tRNA molecule is loaded with a specific amino acid corresponding to its anticodon, which pairs with a matching codon on the mRNA.
The functions of tRNA can be highlighted in the following ways:
- Amino Acid Transfer: tRNA molecules bring amino acids to the ribosome, facilitating the translation of mRNA sequences into polypeptides.
- Anticodon-Codon Matching: The specific pairing between the tRNA anticodon and the mRNA codon ensures the correct amino acids are incorporated into the growing polypeptide chain.
- Adaptability: tRNAs can mobilize quickly during translation, optimizing the use of available amino acids and expediting the synthesis process.
In essence, without tRNA's relentless work, the translation process could not operate smoothly, leading to mishaps in protein assembly.
Regulatory RNA
Regulatory RNA encompasses different types of RNA that influence gene expression without coding for proteins. This group has gained attention for its role in fine-tuning cellular functions and responses. Among these, microRNAs (miRNAs) and long non-coding RNAs (lncRNAs) are particularly noteworthy.
Hereās what you need to know:
- MicroRNAs: These small molecules modulate gene expression by binding to complementary mRNA targets, usually promoting degradation or inhibiting translation.
- Long Non-Coding RNAs: Unlike their smaller counterparts, lncRNAs can regulate gene expression at various levels, including chromatin remodeling and transcriptional interference.
- Role in Cellular Homeostasis: Regulatory RNAs ensure that specific genes are expressed at the right time and in the right amount, acting like conductors in an orchestra to maintain harmony within the cell.
RNA Synthesis: Transcription Process
RNA synthesis, or transcription, lays the groundwork for gene expression. It is the process through which DNA is transcribed into RNA, orchestrating the intricate ballet of cellular functions. Understanding the nuances of this process is crucial for grasping how organisms utilize genetic information. This section explores the initiation, elongation, termination of transcription, and the modifications that RNA undergoes afterward. Each of these steps holds significance in maintaining the delicate balance of cellular activities.
Initiation of Transcription
The initiation stage is like the starting gun at a race; it sets everything in motion. RNA polymerase, the enzyme responsible for synthesizing RNA, first binds to a specific region of the DNA known as the promoter. This region signals to the polymerase where to start reading the DNA sequence. The complexity of this step cannot be overstated, as various transcription factors play roles akin to backstage crew, making sure everything runs smoothly. Once the RNA polymerase is firmly attached, the DNA strand unwinds, setting the stage for RNA synthesis to commence.
It is here that a sort of molecular recognition magic happens. The transcription factors and RNA polymerase ensure that only specific genes are expressed at the right time. If this process fails, it could lead to inefficient cell function or diseases. Itās a bit like trying to start a car with an incompatible key ā nothing happens until the correct match is found.
Elongation and Termination
After initiation, the transcription process shifts gears into elongation. During this phase, the RNA polymerase moves along the DNA, stitching together complementary RNA nucleotides. The elongation phase is where the actual 'building' materializes. The RNA strand grows longer as the polymerase pushes through the DNA, likened to a train barreling down tracks.
However, coherence in this process isn't just about speed; accuracy is vital. RNA polymerase checks for errors but this is augumented by proofreading mechanisms in the cell. Once the RNA strand is assembled, you'll often find it floating free, a new transcript ready for its next role.
The termination phase signals that the transcription journey is nearing its end. Here, specific sequences on the DNA trigger the RNA polymerase to halt its activity. This is much like crossing a finish line but with more intricate details. The termination sequences ensure that the entire gene is accurately transcribed, preventing incomplete RNA from causing downstream issues.
Post-Transcriptional Modifications
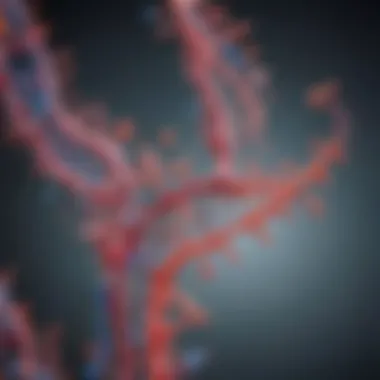
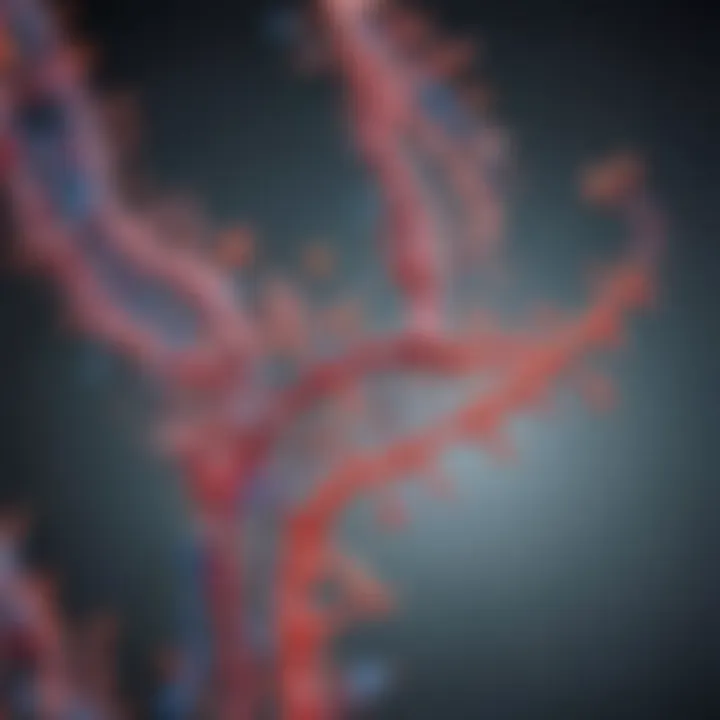
After RNA has been synthesized, it doesn't simply jump from the nucleus into the cytoplasm. Instead, it goes through a series of vital modifications, ensuring itās ready for its roles. These modifications include capping, polyadenylation, and splicing.
- Capping: A 7-methylguanylate cap is added to the 5ā end of the RNA, protecting it from degradation and assisting in the translation process.
- Polyadenylation: A tail of adenine nucleotides, known as the poly-A tail, is added to the 3ā end. This tail plays a role in nuclear export and stability of the RNA.
- Splicing: Introns, the non-coding regions, are removed, while exons, the coding sequences, are joined together. This editing is crucial because it allows for the production of various protein isoforms from a single gene.
These post-transcriptional modifications are absolute necessities for functionality. They not only ensure the integrity of the RNA but also maximize the protein-coding potential of the genetic material. Without these modifications, the synthesized RNA could lead to malfunctioning proteins, akin to trying to read an instruction manual with missing pages.
"The transcription process highlights the elegance of gene regulation, underscoring how DNA translates into lifeās building blocks through a series of precise steps."
In summary, RNA synthesis via transcription is a fundamental process that serves to translate genetic instructions into action. Understanding each stage of this process enlightens the intricate orchestration of cellular life, revealing just how much goes on behind the scenes.
The Role of RNA in Translation
The translation process is a crucial step in the flow of genetic information from DNA to proteins, where ribonucleic acid (RNA) plays a pivotal role. The importance of RNA in this framework cannot be overstated; it functions as the interpreter of the genetic code, ensuring that the right amino acids are assembled in the right order to form functional proteins. Without RNA, the instructions encoded within DNA would remain inert, and cellular machinery would struggle to build the necessary proteins for life.
Codon-Amino Acid Relationship
At the heart of translation lies the codon-amino acid relationship, a specialized pairing system where clusters of three nucleotides, known as codons, correspond to specific amino acids. Each codon on the messenger RNA (mRNA) is read by the ribosome, which looks for matching transfer RNA (tRNA) molecules carrying the appropriate amino acid. This relationship is not random; rather, itās grounded in the genetic code, which dictates that specific codons are linked to specific amino acids. For instance:
- The codon AUG signals the start of protein synthesis and corresponds to Methionine.
- UUU translates to Phenylalanine.
- AUA codes for Isoleucine.
The precision of this relationship ensures that proteins are synthesized accurately, reflecting the original genetic instructions. Any error in this codon-amino acid pairing can lead to dysfunctional proteins, which might cause undetermined cellular issues.
Ribosome Functionality
The ribosome acts as the workbench for translation, composed of rRNA and proteins. Itās here where the magic of translation occurs. Ribosomes can be thought of as molecular machines, tirelessly translating mRNA code into a string of amino acids. They have distinct sites that play different roles:
- A Site (Aminoacyl site): Holds the incoming tRNA with its amino acid.
- P Site (Peptidyl site): Contains the growing protein chain as tRNA carries its respective amino acids.
- E Site (Exit site): Where empty tRNA exits the ribosome after its amino acid has been added to the chain.
As the ribosome moves along the mRNA strand, it facilitates the formation of peptide bonds between amino acids. This multifunctional nature of the ribosome is essential, ensuring that the process of protein synthesis is both swift and accurate, each second producing an ever-growing polypeptide chain.
Peptidyl Transferase Activity
The peptidyl transferase activity is a critical function of the ribosome, responsible for catalyzing the formation of peptide bonds between adjacent amino acids. This enzymatic activity is facilitated by rRNA rather than protein, highlighting the evolutionary importance of RNA in biological processes. Essentially, when the ribosome aligns the tRNA molecules in the appropriate sites, the peptidyl transferase activity links the carboxyl group of one amino acid to the amino group of another, effectively forming a peptide bond. This reaction is a cornerstone of protein synthesis; without it, proteins would not assemble correctly.
Understanding the mechanics of translation, especially the intricate codon-amino acid pairing and ribosomal functionality, provides astounding insights into molecular biology and biotechnology. Cumulatively, these processes underscore the critical roles RNA plays in lifeās foundational structure and function.
Ultimately, the role of RNA in translation encapsulates its versatility and necessity within cellular processes. The seamless interaction between mRNA, tRNA, and ribosomes showcases a sophisticated level of biochemical orchestration that underscores the elegance of life at the molecular level.
RNA as a Regulator of Gene Expression
RNA is more than just a messenger in the grand symphony of genetic expression; it acts as a conductor, directing and fine-tuning the biological orchestra that is our cells. The role of RNA in regulating gene expression is a topic that deserves our utmost attention. In recent decades, researchers have illuminated how various types of RNA influence when and how genes are expressed, which is a pivotal element not only in developmental biology but also in understanding diseases.
Through mechanisms like gene silencing and feedback loops, RNA has shown itself to be a master regulator. This section discusses the critical elements and implications of RNA as a regulator of gene expression, elaborating on the specific roles of microRNAs, long non-coding RNAs, and feedback mechanisms. Understanding these processes can offer insights that are fundamental to advancements in fields like medicine and biotechnology.
MicroRNAs and Gene Silencing
MicroRNAs (miRNAs) are short, non-coding RNA molecules typically about 20-22 nucleotides long. They are often considered the unsung heroes of gene regulation. Originating from longer RNA precursors, these miRNAs undergo processing before they become functional. Once active, they can bind to complementary sequences within mRNA, leading to targeted gene silencing.
This silencing can occur through translational repression or mRNA degradation, effectively lowering the expression levels of specific genes. This mechanism plays a crucial role in controlling cellular processes such as proliferation, differentiation, and apoptosis. Furthermore, the dysregulation of miRNA pathways has been linked to various diseases, including cancer, highlighting their significance.
For instance, certain miRNAs can act to suppress oncogenes, those genes that can promote cancer when altered or expressed at high levels. They can act like brakes on cellular growth, which shows us just how finely tuned these regulatory networks can be. A failure in this balance could allow uncontrolled cell growth, paving the way for tumors.
"MicroRNAs serve as crucial checkpoints in gene regulation, reminding us how nuanced biology can be."
Long Non-Coding RNAs
Long non-coding RNAs (lncRNAs), which are longer than 200 nucleotides, circle back to the orchestra metaphor by playing supporting roles that can orchestrate a variety of functions within the cell. Unlike miRNAs, they donāt necessarily silence genes but can influence the expression and chromatin dynamics in complex ways.
LncRNAs can interact with both DNA and proteins, thus modulating gene expression at multiple levels. They can serve as scaffolds, bringing together various proteins to form regulatory complexes or even guiding transcription factors to specific gene loci. Some lncRNAs play crucial roles in maintaining stem cell identity, while others are implicated in processes like X-chromosome inactivation.
A tangible example includes XIST, a well-studied lncRNA essential for X-inactivation in female mammals. When expressed, XIST coats one of the X chromosomes, leading to its silencing. The very existence of such mechanisms suggests that lncRNAs contribute widely to the complexities of cellular differentiation and function.
Feedback Mechanisms
Feedback mechanisms involving RNA provide deeper insights into how gene expression is modulated. These mechanisms can be positive or negative, serving to amplify or dampen signaling pathways. For instance, in certain pathways, increasing levels of a protein might upregulate the corresponding mRNA, resulting in more protein production.
Conversely, negative feedback mechanisms often involve regulatory RNA like miRNAs or lncRNAs. Here, increased expression of a target protein might elevate the levels of an opposing miRNA, which in turn reduces further protein production. Such practices ensure that cellular activities remain finely balanced, akin to tuning an instrument to achieve the perfect sound.
These feedback loops play a crucial role in processes like hormonal signaling and metabolic regulation. Misadjustment in these systems can lead to various pathologies, underscoring the importance of RNA in maintaining homeostasis within biological systems.
RNA's Role in Viral Infections
The interplay between RNA and viral infections has become an essential aspect of understanding how various viruses operate within hosts. RNA viruses, in particular, are a major concern in virology and medicine due to their capacity for rapid replication and mutation. Importantly, many viruses utilize RNA as their genetic material, which sets the stage for direct interaction with the host's cellular machinery. In this section, we'll explore two primary facets of this relationship: the unique structures of viral RNA and the processes involved in replication within host cells.
Viral RNA Structures
Viral RNA structures are diverse and tailored intricately to optimize their infectivity and replication potential. Unlike the stable double-helix structure of DNA, viral RNA can exist in various configurations, such as single-stranded or double-stranded forms. These configurations are often crucial for the virus's infective capabilities.
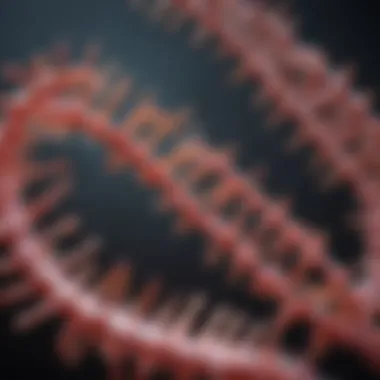
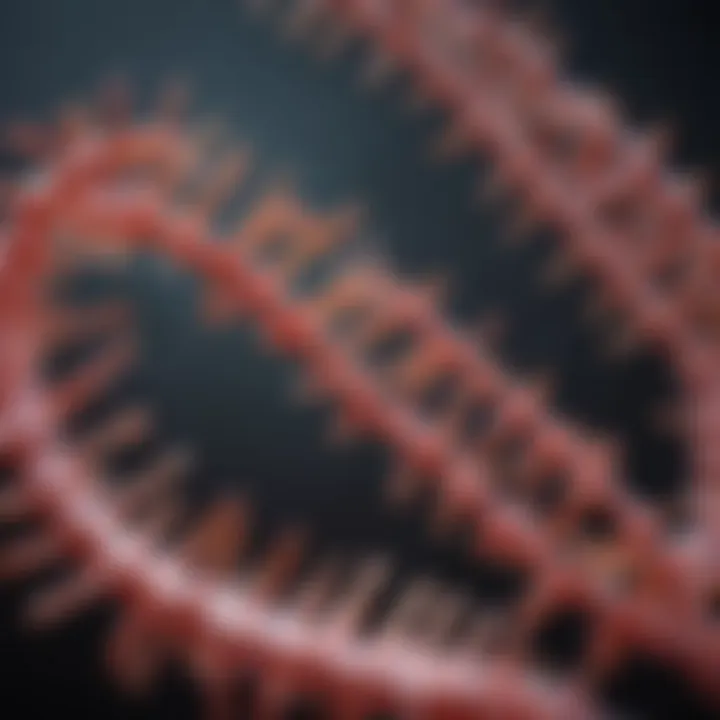
For instance, some viruses, like influenza, possess segmented RNA, which allows them to easily exchange genetic material during co-infection of a host cell. This feature enhances genetic diversity and adaptability, contributing to difficulties in vaccine development. In contrast, other viruses, such as poliovirus, utilize a single-stranded positive-sense RNA, which can be directly translated into proteins upon entering a host cell.
It's also worth noting that the structures of these viral RNAs often include specific sequences known as RNA motifs. These motifs play critical roles in processes such as translation initiation, packaging, and interaction with host factors. Notably, the secondary structures formed by viral RNA can influence the virus's ability to evade the host's immune response. Thus, understanding viral RNA structures not only highlights their uniqueness but also emphasizes their functional significance in viral infectivity.
Replication in Host Cells
Once inside a host cell, viral RNA replication follows a series of well-orchestrated steps that leverage the host's cellular machinery. This process typically begins when the viral RNA is released into the cytoplasm following uncoating. The host's ribosomes then recognize the viral RNA and commence translation of viral proteins necessary for replication.
During replication, RNA viruses often employ two key strategies:
- Positive-sense RNA viruses: They can immediately act as messenger RNA (mRNA), leading to the direct synthesis of viral proteins. The replication involves the synthesis of a complementary negative-sense RNA strand, which then serves as a template for producing more positive-sense RNA.
- Negative-sense RNA viruses: These viruses must first be converted into positive-sense RNA by an enzyme called RNA-dependent RNA polymerase, which they bring along in their viral particle. After this conversion, the positive-sense RNA can be translated into proteins, and more negative-sense RNA strands can be synthesized.
The efficiencies of these replication strategies can significantly impact the severity and spread of viral infections. Moreover, certain errors in replication can lead to mutations, potentially resulting in new viral strains that may evade the immune system or resistance strategies.
"Understanding the mechanisms of RNA virus replication is a cornerstone in developing therapeutic interventions and vaccines that can combat viral diseases effectively."
RNA in Biotechnology
Ribonucleic acid (RNA) has emerged as a cornerstone in the field of biotechnology, serving numerous functions that underscore its significance in modern scientific advancements. From its instrumental role in gene expression to its utilization in novel therapeutic strategies, RNA stands at the forefront of innovations that push the boundaries of our understanding and capabilities. The transition from traditional genetic methods to RNA-based approaches has opened doors to a multitude of applications, highlighting the growing relevance of RNA in various biotechnological endeavors.
RNA Interference in Genetic Engineering
One of the most powerful tools harnessed from RNA is the technique of RNA interference (RNAi). This natural cellular process allows for the silencing of specific genes, offering researchers a precise mechanism to modulate gene expression. RNAi is typically facilitated by small interfering RNAs (siRNA) or microRNAs (miRNA), which bind to target messenger RNA (mRNA) molecules, leading to their degradation or preventing their translation into proteins. The benefits of RNAi in genetic engineering are manifold:
- Targeted Gene Silencing: This enables researchers to investigate gene function by observing the effects of silencing specific genes in an organism or cell line.
- Therapeutic Applications: RNAi has potential therapeutic uses in treatments for diseases caused by overactive genes or genetic disorders. Developing RNAi-based drugs shows promise in areas such as cancer therapy, where the modulation of oncogenes can inhibit tumor growth.
- Agricultural Improvements: In agriculture, RNAi can be employed to develop genetically modified crops with enhanced resistance to pests or diseases, thereby increasing yield and reducing pesticide use.
However, while the potential is vast, there are important considerations regarding the delivery and specificity of RNAi mechanisms. The challenge lies in ensuring that RNAi agents effectively reach target cells without unintended side effects or off-target gene silencing, which could lead to unforeseen consequences.
RNA Therapeutics
RNA also plays a crucial role in the burgeoning field of therapeutics. As science has delved deeper into the understanding of RNA's functions, it has paved the way for RNA-based therapeutics that offer promising avenues for treatment. These can be categorized into several noteworthy approaches:
- M RNA Vaccines: Perhaps most famously exemplified by the quick development of COVID-19 vaccines, mRNA vaccines utilize strands of mRNA to instruct cells to produce proteins that trigger an immune response, providing immunity against pathogens without the use of live virus.
- Antisense Oligonucleotides: These are short, single-stranded pieces of nucleic acid that bind to mRNA, leading to its degradation or preventing translation. They offer treatment possibilities for various genetic disorders, including Duchenne muscular dystrophy and spinal muscular atrophy.
- Gene Therapy: RNA therapies can also be employed to replace or compensate for defective genes by delivering functional versions of RNA directly to affected cells.
The versatility of RNA as a therapeutic agent highlights its transformative potential in medicine. However, with great promise comes the need for thorough exploration of safety assessments, potential immune responses, and long-term effects of RNA therapies in patients.
The integration of RNA technologies into biotechnology represents a paradigm shift in how we approach medical and agricultural challenges. This innovation not only enriches our understanding of life at the molecular level but also equips us with powerful tools for the future.
Recent Advances in RNA Research
In the ever-changing world of molecular biology, RNA research has taken bold strides forward, uncovering nuances that were once shrouded in mystery. The exploration of RNAās intricacies not only enhances our understanding of cellular biology but also opens doors to new therapeutic avenues. This section dives into recent advancements, highlighting their significance and the implications they hold for science and medicine.
Next-Generation Sequencing Technologies
Next-generation sequencing (NGS) has revolutionized RNA research. With its ability to sequence millions of fragments of RNA simultaneously, NGS allows scientists to gain insights into the RNA landscape of various organisms at an unprecedented scale. Here are a few key aspects of NGS that have significantly impacted RNA studies:
- Speed and Efficiency: Compared to traditional methods, NGS is remarkably swift, generating data that was once deemed unattainable in a reasonable timeframe. This allows researchers to move faster through the research process.
- High Throughput: The capability to analyze numerous samples in one go means that large-scale experiments can be executed without the bottleneck of previous techniques.
- Unearthing Novel RNA Species: NGS has facilitated the discovery of thousands of new non-coding RNAs, helping illuminate their functions and roles in different biological processes.
With these tools at hand, scientists are not just cataloguing RNA; they are decoding the very scripts that dictate cellular behavior.
RNA Modifications and Their Implications
RNA is not a static molecule; it undergoes various modifications that can greatly affect its function. The study of these modifications has led to new insights regarding gene expression and cellular response mechanisms. Hereās a closer look at what these modifications are and why they matter:
- Types of RNA Modifications:
- Biological Significance:
- Research and Future Directions:
- Methylation: This addition transforms RNA, impacting its stability and interaction with proteins. Methylated RNA can escape degradation, prolonging its functional life.
- Pseudouridylation: This modification can alter RNA structure, influencing its role in translation and regulation.
- These modifications are not just decorative. They play critical roles in regulating gene expression and guiding protein synthesis. For instance, properly modified RNA can enhance translation efficiency, ensuring that proteins are produced at the right time and in the right amounts.
- In some cases, the absence or alteration of specific modifications is linked to diseases, hinting at potential therapeutic targets for interventions.
- Understanding how these modifications affect cellular mechanisms could pave the way for novel RNA therapies, including precision medicine that tailors treatments based on an individualās RNA profile.
"The future of RNA research lies in the nuanced understanding of its modifications and how they orchestrate life processes at a molecular level."
By staying abreast of these developments, researchers aim not only to grasp the fundamental principles of RNA function but also to harness this knowledge for innovative therapeutic strategies.
Ending: The Ever-Evolving Role of RNA
Ribonucleic acid (RNA) serves as a cornerstone in the understanding of molecular biology and genetics. Its dynamic nature keeps researchers on their toes, constantly discovering new roles and functions that challenge our previous notions. The exploration of RNA is not just an academic exercise; it holds profound implications for medicine, biotechnology, and our general grasp of the life sciences.
The Importance of RNA in Contemporary Science
From being long perceived mainly as a messenger between DNA and proteins, RNA's relevance has burgeoned. Researchers have identified various types of RNA, such as microRNAs and long non-coding RNAs, that possess sophisticated regulatory functions. This nuanced understanding shifts the focus from simple genetic coding to the broader spectrum of gene expression, regulation, and cellular dynamics. The implications are significant in understanding diseases at a molecular level.
Benefits and Considerations
- Disease Understanding & Treatment
The continuous advancement of RNA research has opened avenues for targeted therapies, especially in areas like cancer, where RNA interactions can directly affect treatment outcomes. - Biotechnology and Genetic Engineering
RNA interference techniques show promise in managing gene expression, leading to innovations in genetic engineering that could potentially cure genetic disorders! - Molecular Pathways
As more is uncovered about how RNA integrates with various cellular pathways, the potential to manipulate these processes expands, which could revolutionize how we approach health and illness.
In an age where genomic information is pivotal, the journey to understand RNA is far from over. With continual advancements in sequencing technologies and analytical methods, the doors are wide open for future discoveries.
"The exploration of RNA is more than just a study of a molecule; itās an odyssey into the fundamental blueprint that dictates life itself."
Summary of Key Points
- RNA is no longer seen merely as a messenger; it has diverse and intricate roles.
- Recent findings highlight its importance in gene regulation and expression.
- Innovations in RNA research hold immense promise, especially in therapeutic applications.
- A deeper understanding of RNA aids in broadband education about diseases and genetic fidelity.
The pathways that RNA opens are intriguing and vibrant. The more we know, the better prepared we are to harness that knowledge for the future.