In-Depth Examination of Kinetics Equations
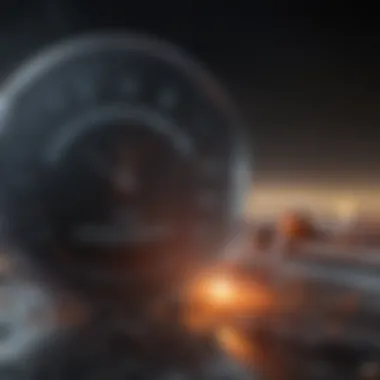
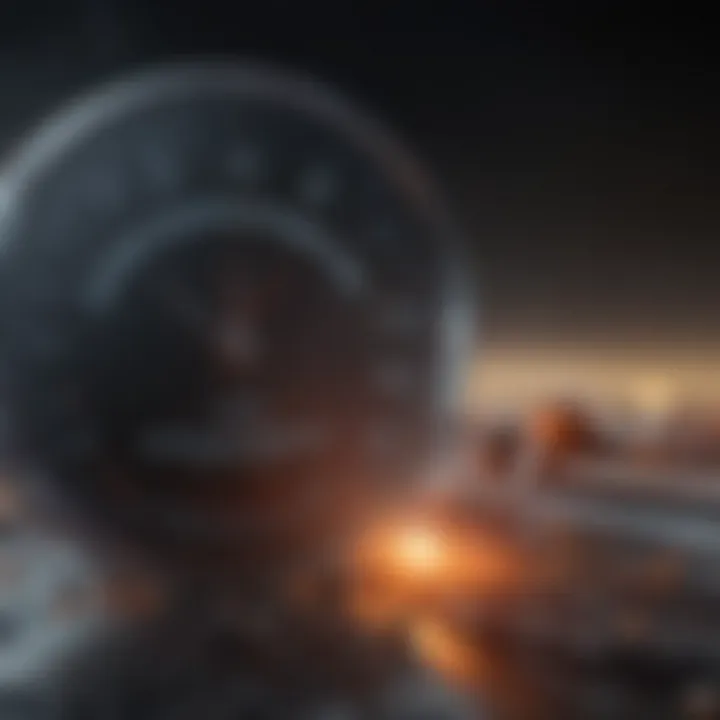
Intro
Kinetics equations are pivotal in the study of how chemical reactions progress over time. Understanding the rates of these reactions forms the cornerstone of fields like physical chemistry, biochemistry, and environmental science. This article seeks to illuminate the various kinds of kinetics equations that are essential for grasping reaction dynamics. In doing so, both students and professionals can enhance their comprehension of reaction mechanisms and their practical outcomes.
The article delves into important concepts such as zero-order, first-order, and second-order reactions, providing a solid foundation for future inquiry. It also examines how temperature and catalysts influence reaction rates, offering insights into their vital roles in industrial processes and environmental management. With a careful integration of theory and real-world applications, we aim to provide a holistic view of the effects of kinetics in diverse scenarios.
Understanding these principles can help improve efficiency and effectiveness in various fields, from pharmaceuticals to manufacturing. Thus, it is clear that a deep knowledge of kinetics equations is essential for scientific progress and innovation.
Intro to Kinetics Equations
Chemical kinetics, or the study of reaction rates, is a vital branch of physical chemistry. Understanding how reactions progress allows scientists to predict outcomes and improve processes. Kinetics equations form the backbone of this discipline, providing quantitative measures that elucidate the speed at which reactions occur.
Importance of Kinetics
Kinetics equations help to uncover fundamental behaviors in chemical reactions. For students and researchers alike, this insight fosters a greater appreciation of reaction dynamics, reaction mechanisms, and how external factors influence these processes. The value of kinetics extends beyond personal academic pursuits; it encompasses industrial applications, environmental assessments, and biological systems.
Key Elements
The first aspect to consider when discussing kinetics equations is the ability to define and measure reaction rates accurately. A well-developed understanding of how reactions proceed over time paves the way for advancements in fields like pharmaceuticals, energy production, and environmental science. Additionally, each type of reaction—zero-order, first-order, and second-order—presents unique characteristics that can be exploited for specific practical applications.
Current Considerations
When delving into kinetics equations, certain factors should be kept in mind. Precision in experimental design is crucial for collecting reliable data. Also, the computational analysis of kinetics equations is becoming increasingly sophisticated, allowing for simulations and predictions that were once unattainable. Moreover, the integration of temperature and catalysis in understanding kinetics increases the complexity of the equations involved and their applications.
"Understanding kinetics provides a roadmap to enhancing reaction efficiency, optimizing yield, and minimizing waste in industrial processes."
In summary, the study of kinetics equations is essential for maximizing our understanding of chemical reactions. As we proceed through this discourse, we will explore the historical development, fundamental concepts, and various types of kinetics equations, gaining insight into their real-world significance and applications. This foundation will better equip students, educators, and professionals aiming to engage more deeply in the science of kinetics.
Historical Background
Understanding the historical background of kinetics equations provides a vital perspective on how these concepts evolved over time. The study of kinetics enables scientists to comprehend the intricacies of reaction rates, which is critical for advancing fields like chemistry, biochemistry, and engineering. Historical insights into kinetics also reveal the progression of scientific thought, demonstrating how theories transitioned from rudimentary ideas to sophisticated models that accurately describe reaction dynamics.
The Beginnings of Kinetic Theory
The inception of kinetic theory can be traced back to the early 19th century. This theory aimed to explain how the motion of particles affects the properties of gases. Pioneers like John Dalton and Amedeo Avogadro laid the groundwork by introducing concepts such as the atomic theory and the idea that gas properties are influenced by particle behavior. These early developments were essential in establishing a scientific framework for understanding reaction rates.
In 1857, Rudolf Clausius further advanced kinetic theory by relating the macroscopic properties of gases to their microscopic activities. This marked a significant milestone, as Clausius's work gave birth to the idea that temperature is a measure of the average kinetic energy of particles. His findings paved the way for a deeper understanding of how temperature influences reaction velocities, which is a central theme in chemical kinetics today.
Key Contributors to Kinetics
The field of kinetics has been shaped by numerous key contributors over the years. Notable figures include:
- Svante Arrhenius: He is renowned for formulating the Arrhenius Equation, which demonstrates the relationship between temperature and reaction rate. His insights into activation energy helped clarify why certain reactions proceed more rapidly at increased temperatures.
- William H. W. M. H. Arrhenius: His work provided clarity to the concept of activation energy, emphasizing its role in predicting reaction dynamics.
- Michael Faraday: Faraday's experiments brought to light the importance of concentration and surface area in reaction rates. His contributions are foundational in the understanding of reactive systems.
These scientists, among others, constructed the theoretical underpinnings of kinetics. Their collective efforts have allowed researchers to analyze reactions with greater precision. The historical evolution of kinetics underscores the importance of collaboration and innovation in scientific discovery.
The development of kinetics is a testament to the spirit of inquiry and the relentless pursuit of understanding the natural world.
By recognizing the historical context of kinetics, readers can better appreciate the theories and equations that drive current research and applications in various fields. The history not only showcases the evolution of thought but also highlights the significance of foundational knowledge in the contemporary study of reaction rates and dynamics.
Fundamental Concepts of Chemical Kinetics
Understanding the fundamental concepts of chemical kinetics is crucial for anyone studying reaction rates in chemistry. Kinetics provides insight into how reactions proceed, how quickly they happen, and the factors that can affect their speed. These concepts lay the groundwork for more advanced theories and applications. Grasping basic principles enables students and professionals to evaluate and predict the behavior of chemical reactions in various conditions, which is essential in fields like pharmaceuticals, industrial processes, and environmental science.
Defining Reaction Rates
Reaction rates are a measure of how fast reactants are converted into products during a chemical process. This definition is simple, yet it encompasses a wide range of phenomena. Reaction rates can be affected by several factors, including concentration, temperature, and the presence of a catalyst. A fundamental understanding of how to define and measure these rates is vital.
One common way to express reaction rates is through the change in concentration of a reactant or product per unit time. This can be mathematically represented as:
where ([A]) refers to the concentration of reactant A, and ([B]) refers to the concentration of product B. The negative sign indicates that the concentration of the reactant decreases over time, while the product concentration increases.
Different reactions exhibit varying rates, influenced by multiple parameters. This makes understanding reaction rates a foundational component of chemical kinetics.
Factors Influencing Reaction Rates
Several critical factors can influence reaction rates. Understanding these elements helps in controlling and optimizing reactions. Some of the key factors include:
- Concentration of Reactants: Generally, an increase in the concentration of reactants leads to a higher reaction rate. This is due to an increased probability of colliding particles.
- Temperature: Higher temperatures typically increase reaction rates. This is due to the greater energy of particles, which leads to more frequent collisions and higher chances of overcoming the activation energy barrier.
- Catalysts: The introduction of a catalyst can significantly alter the rate of a reaction. Catalysts work by lowering the activation energy required for a reaction to occur, hence accelerating the process.
- Surface Area: For solid reactants, increasing surface area can enhance reaction rates. Finely divided solids have more surface area exposed for reaction compared to larger pieces.
- Nature of the Reactants: The specific chemical properties of the reactants involved also dictate reaction rates. Reactions involving ionic species often occur more rapidly than those involving covalent bonds.
Understanding these influencing factors is essential for scientists and researchers. It not only aids in predicting reaction behavior under different conditions but also guides experimental design and practical applications in various fields.
The study of reaction rates and their influencing factors is fundamental to comprehending how reactions are controlled and manipulated in industrial processes and biological systems.
Types of Kinetics Equations
Understanding the various types of kinetics equations is essential for grasping the behavior of chemical reactions over time. Each category of reaction reveals different insights into how substances interact, change, and evolve during a reaction. The importance of differentiating between these types lies in their distinct mathematical descriptions and applicability to real-world situations. Recognizing the differences provides chemists with tools to predict outcomes, optimize processes, and understand underlying mechanisms.
Benefits of Understanding Types of Kinetics Equations:
- Enables accurate modeling of reactions.
- Facilitates the prediction of reaction behaviors under varied conditions.
- Aids in optimizing industrial processes by selecting the appropriate reaction type for efficiency.
Key Considerations:
- Each type of reaction may behave differently depending on external conditions like temperature and concentration.
- Misinterpretations of kinetics can lead to ineffective experimental designs or industrial applications.
Zero-Order Reactions
Zero-order reactions are characterized by a constant rate that is independent of the concentration of reactants. This means that the reaction proceeds at a steady rate until the reactants are depleted. Such reactions often occur in systems where one reactant is in excess, such as in enzyme saturation scenarios in biochemical reactions.
The rate law for a zero-order reaction can be expressed as:
[
extRate = k
]
Where (k) is the zero-order rate constant.
Key Features:
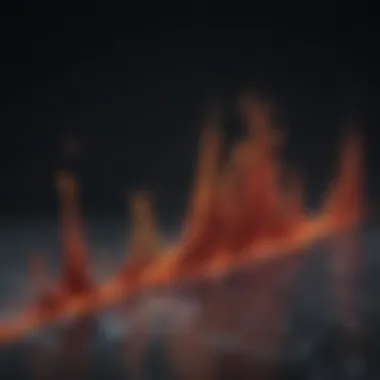
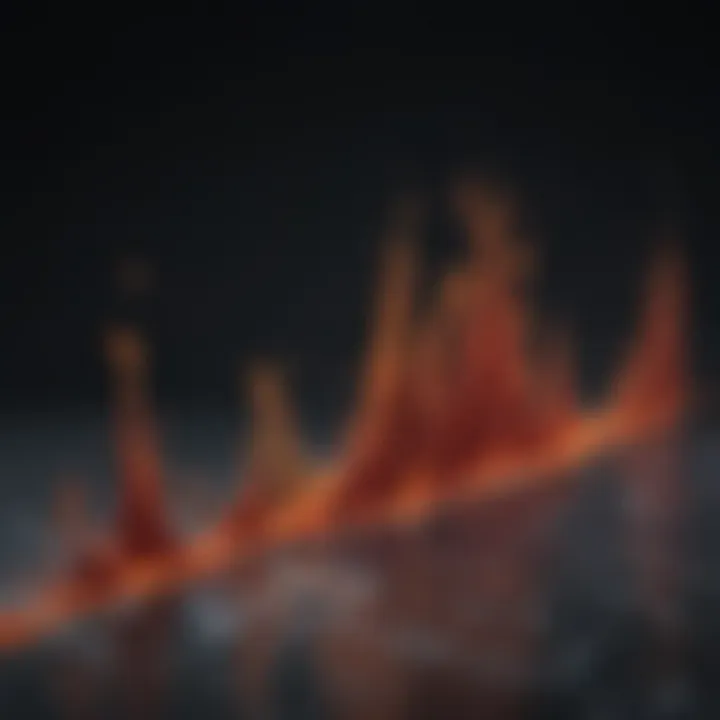
- Rate Independence: The reaction rate remains constant.
- Integration: The concentration of the reactant decreases linearly over time.
First-Order Reactions
First-order reactions depend linearly on the concentration of a single reactant. The rate of reaction accelerates as the reactant concentration increases. This type of kinetics is common in radioactive decay and simple unimolecular reactions.
The rate law is given by:
[
extRate = k[ extA]
]
Where ([ extA]) denotes the concentration of reactant A.
Characteristics:
- Exponential Behavior: The concentration decreases exponentially over time.
- Half-Life: The half-life of a reaction is constant and independent of initial concentration, which is a unique property of first-order reactions.
Second-Order Reactions
Second-order reactions can either involve two reactants or a single reactant in a squared term. The reaction rate depends on the concentration of reactants raised to the second power.
The rate law can be defined as:
[ extRate = k[ extA]^2 ]
or
[
extRate = k[ extA][ extB]
]
Distinguishing Traits:
- Concentration Dependency: The rate of the reaction substantially changes as the concentration varies.
- Integration: The concentration decrease is quadratic, resulting in a more complex behavior compared to zero and first-order reactions.
Pseudo-Order Reactions
Pseudo-order reactions arise when one of the reactants is in large excess. This causes the reaction to behave as if it is a first or second order even when more reactants are involved. For instance, in a reaction involving a solvent in much larger amounts, the concentration of the solvent can be considered constant.
While the true order of the reaction may be higher, the reaction can be simplified for analysis.
Representations:
- This simplification allows the use of first or second-order kinetics to describe reactions effectively while ignoring the excess reactant changes.
Understanding these distinctions plays a crucial role in chemical kinetics, allowing for effective experimentation and application across various scientific fields.
Mathematical Formulations
Mathematical formulations are critical to understanding kinetics equations, providing a structured method to analyze reaction rates and predict the outcomes of chemical processes. They are rooted in observable data and help scientists develop models that reflect the real behavior of chemical systems. Such structured approaches enhance precision in evaluating how reaction conditions affect outcomes. The utility of mathematical formulations lies in their ability to distill complex reactions into understandable equations, thereby facilitating easier interpretation and practical application.
Derivation of Rate Laws
The derivation of rate laws is foundational in kinetics. A rate law expresses the relationship between the rate of a chemical reaction and the concentration of its reactants. Understanding this linkage is pivotal for predicting how changes in concentration can influence reaction speed.
When deriving a rate law, empirical observations dictate how concentration affects reaction rate, leading to a general form:
Rate = k [A]^m [B]^n
Here, is the rate constant, and and represent the concentrations of reactants A and B, respectively, while and denote the reaction orders relating to each reactant. This formulation enables chemists to quantify the reaction dynamics.
Another crucial aspect is determining the values of and , which can only be determined experimentally. Various methods, such as the method of initial rates or integrated rate laws, can be used. Ultimately, the derived rate laws illuminate the complex interactions among reactants and conditions influencing their reactions.
Integrated Rate Laws
Integrated rate laws provide a means to relate concentration changes over time, allowing for a deeper understanding of the kinetics of reactions. While rate laws describe the instantaneous rate, integrated forms reveal how concentrations change as reactions progress.
Different reaction orders yield distinct integrated rate laws. For example:
- Zero-order reactions:
[A] = [A]₀ - kt
In this case, concentrations decrease linearly with time. - First-order reactions:
ln[A] = ln[A]₀ - kt
Here, the logarithmic relationship holds, indicating an exponential decay of concentration. - Second-order reactions:
1/[A] = 1/[A]₀ + kt
This characterizes a quadratic relationship over time.
These equations form a bridge between experimental data and theoretical understanding, enabling precise predictions about reaction behavior. Utilizing integrated rate laws assists chemists not just in analyzing past reactions but also in predicting future behaviors in varied contexts, from industrial applications to research initiatives.
The Role of Temperature in Kinetics
Temperature plays a pivotal role in determining the rate of chemical reactions. Understanding its influence is crucial for anyone studying kinetics. As temperature increases, the kinetic energy of molecules also rises. This results in more frequent and energetic collisions between reactants, which directly affects the speed of reactions. A thorough examination of this relationship helps reveal critical insights into reaction mechanisms and their efficiency.
One of the foundational concepts in relating temperature to reaction rates is captured by the Arrhenius Equation. The Arrhenius Equation mathematically expresses how the rate constant, k, varies with temperature. It is given by:
where:
- k is the rate constant,
- A is the frequency factor or pre-exponential factor,
- E_a is the activation energy,
- R is the universal gas constant, and
- T is the absolute temperature in Kelvin.
This equation demonstrates that as temperature increases, the exponential term becomes larger. This indicates that the rate constant k increases, leading to faster reaction rates. The Arrhenius Equation is central to many studies in chemical kinetics, illustrating the profound impact of temperature on reaction dynamics across various scientific fields.
Arrhenius Equation
The Arrhenius Equation serves as a cornerstone for kinetics studies, linking temperature to the activation energy of a reaction.
- Activation energy (E_a) is the minimum energy required for a reaction to occur.
- Frequency factor (A) accounts for the factors that contribute to the frequency of collisions between reactants.
By studying the Arrhenius Equation:
- Chemical engineers can efficiently tailor processes that require specific temperatures.
- Researchers can identify potential energy barriers in biochemical reactions.
It is worth noting that the Arrhenius Equation is not just theoretical. It has practical implications across various fields, from industrial chemistry to biochemistry, making it indispensable for understanding kinetics.
Temperature Dependence of Rates
The dependence of reaction rates on temperature is crucial for both theoretical and practical applications. As established earlier, an increase in temperature leads to an increase in reaction rates. Some key points to consider include:
- Rate Increase: Many reactions show a significant increase in rate with temperature. Generally, a temperature rise of 10 °C can often double the reaction rate for many reactions.
- Variability Across Reactions: Not all reactions respond equally to temperature changes. Some reactions exhibit a more pronounced sensitivity, making specific study essential for each case.
- Thermodynamic Considerations: Higher temperatures can lead to changes in equilibrium positions in reversible reactions. A comprehensive understanding of the enthalpy and entropy changes involved is necessary for a complete analysis.
In summary, the role of temperature in kinetics cannot be overstated. It is essential for understanding how reactions proceed in various conditions, influencing both the theoretical framework and practical applications. Understanding the Arrhenius Equation and the temperature dependence of reaction rates enables chemists, engineers, and biologists to optimize reaction conditions and predict outcomes accurately.
Catalysis and Kinetics
Catalysis plays an important role in kinetics, significantly influencing the rates of chemical reactions. It helps streamline reactions that would otherwise proceed too slowly to be practical. In this section, we will examine both homogeneous and heterogeneous catalysis, as well as the mechanisms involved in catalytic reactions. Understanding these concepts is crucial for various applications, from industrial processes to environmental management.
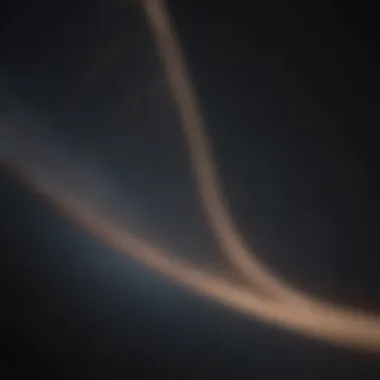
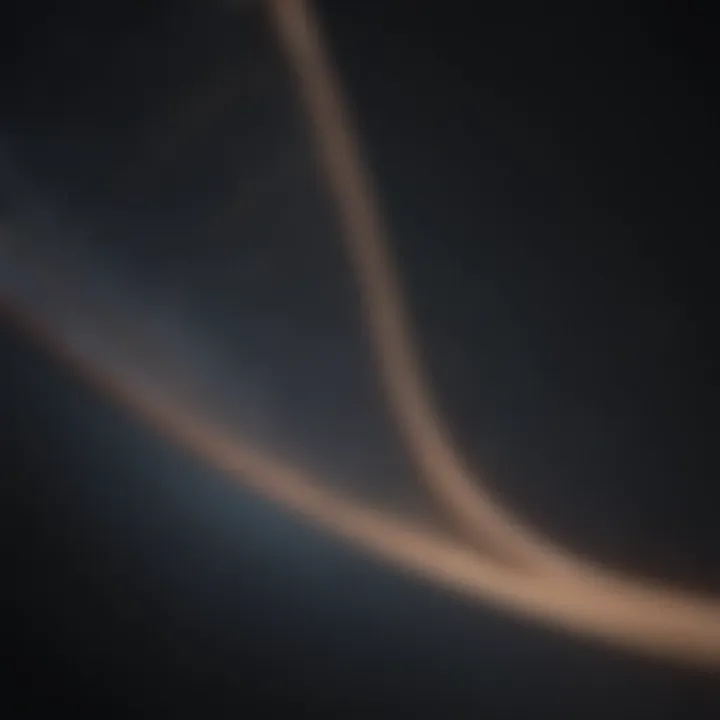
Homogeneous vs Heterogeneous Catalysis
Homogeneous catalysis occurs when the catalyst and the reactants are in the same phase, usually in a solution. An advantage of this type of catalysis is the uniform interaction between the reactants and the catalyst, which can enhance reaction efficiency. A common example is the use of sulfuric acid in the esterification reaction, where the acid is mixed with the reactants in the liquid state.
In contrast, heterogeneous catalysis involves a catalyst in a different phase than the reactants, commonly a solid catalyst with gaseous or liquid reactants. This type of catalysis often allows for easier separation of the catalyst from products. Catalysts like platinum or palladium in catalytic converters for vehicles provide a practical illustration. During the reaction, gaseous pollutants interact with the solid catalyst, converting harmful substances into less harmful emissions.
Key Differences:
- Phase: Homogeneous catalysts are in the same phase as reactants; heterogeneous catalysts are in a different phase.
- Interaction Efficiency: Homogeneous offers better mixing and interaction; heterogeneous provides easier catalyst recovery.
Mechanisms of Catalytic Reactions
Catalytic reactions can follow various pathways depending on the nature of the catalyst and the reactants. Understanding the mechanisms is essential for developing more efficient catalytic processes. The elementary steps in these mechanisms typically include adsorption, where reactants adhere to the surface of the catalyst; reaction, where the actual transformation occurs; and desorption, where products leave the catalyst's surface.
- Adsorption: Reactants bind to the catalytic surface, forming an intermediate complex.
- Reaction: This complex undergoes transformation into products.
- Desorption: The products detach from the catalyst surface, making space for more reactants.
An important aspect to consider is the factors influencing the efficiency of these mechanisms, such as surface area, temperature, and the presence of inhibitors. For instance, if the catalyst's surface area is increased, reactions often occur at higher rates due to more available active sites. Similarly, temperature variations can change reaction rates significantly, as explained by the Arrhenius equation.
The efficiency of a catalyst not only depends on the material used but also on the specific reaction conditions applied.
By studying both homogeneous and heterogeneous catalysis, researchers can optimize reaction conditions and enhance the overall efficiency of kinetic processes across both laboratory and industrial settings.
Applications of Kinetics Equations
The applications of kinetics equations extend across various fields, underscoring their significance in both theoretical understanding and practical utility. Kinetics helps decipher the complexities of how substances interact over time and provides insights into optimizing various processes. Understanding the dynamics behind reaction rates is essential for innovation and efficiency in multiple domains.
Industrial Reactions
In industries, kinetics equations play a pivotal role. They guide the design and optimization of reactors, ensuring that chemical transformations occur efficiently. By applying zero-order, first-order, and second-order kinetics, engineers can predict how changes in concentration, temperature, or pressure affect reaction rates.
For instance, the Haber process for synthesizing ammonia profoundly relies on kinetics. By optimizing the conditions based on reaction kinetics, the yield can be significantly improved. Kinetics also aids in scaling up reactions from laboratory settings to industrial applications. Companies like BASF and DuPont utilize kinetic models to refine their processes, resulting in cost savings and minimized waste.
Key benefits of applying kinetics in industrial settings include:
- Enhanced efficiency of chemical processes.
- Reduction in production costs.
- Improved safety by predicting potential hazards related to reaction rates.
- Ability to design continuous reactors that operate under optimal conditions.
Environmental Impact
Kinetics equations are equally vital in the environmental realm. They help in understanding the degradation processes of pollutants, influencing strategies for waste management and remediation. The rates of reaction for carbon compounds in the atmosphere, for instance, are influenced by temperature and the presence of catalysts. Such understanding is pivotal in combatting climate change and reducing emissions.
The influence of kinetics on environmental applications can be summarized as follows:
- Predicting the behavior of pollutants in natural systems.
- Formulating effective strategies for bioremediation, which relies on understanding how microbes degrade contaminants.
- Evaluating the impact of chemicals on ecosystem health through rates of biodegradation.
"Understanding reaction kinetics is crucial for predicting the lifetimes and impacts of pollutants in the environment."
By studying kinetics in relation to environmental concerns, researchers can develop better regulations and approaches to protect natural resources and enhance public health.
In closing, the applications of kinetics equations serve not only as a foundation for scientific inquiry but also as practical tools for industry and environmental management. Their importance cannot be overstated, as they bridge theoretical knowledge with real-world applications.
Kinetics in Biological Systems
Kinetics in biological systems emphasizes the significance of reaction rates in the complex biochemical processes that occur in living organisms. This area of study is essential as it helps us understand how various factors influence enzymatic reactions and metabolic pathways. Such understanding has far-reaching implications, from drug development to the advancement of biotechnological applications.
Enzyme Kinetics
Enzyme kinetics focuses specifically on the rates of enzyme-catalyzed reactions. Enzymes are biological catalysts that accelerate chemical reactions without being consumed. Understanding enzyme kinetics allows scientists to determine how quickly a reaction occurs under specific conditions.
The study often employs the Michaelis-Menten model, which delineates the relationship between substrate concentration and the reaction rate. Key parameters in enzyme kinetics include:
- Vmax: The maximum rate of reaction when the enzyme is saturated with substrate.
- Km: The substrate concentration at which the reaction rate is half of Vmax.
These parameters provide insights into enzyme efficiency and its affinity for the substrate, which are crucial for drug design and therapeutic interventions. The reactonal rates can be influenced by temperature, pH, and the presence of inhibitors or activators, making it important to study these factors for better applications in biology and medicine.
Metabolic Pathways
Metabolic pathways represent a series of interconnected biochemical reactions that convert one substance into another within the cell. Understanding the kinetics of these pathways is vital for several reasons.
- Homeostasis: Kinetics helps in maintaining a stable internal environment by regulating flux through metabolic pathways.
- Disease Mechanisms: Anomalies in reaction rates can lead to metabolic disorders. Identifying these irregularities can inform medical treatments.
- Biotechnological Advances: Knowledge of kinetics aids in optimizing conditions for biotechnological applications, like fermentation or bioremediation.
The study of metabolic pathways often involves detailed kinetic modeling to simulate reaction dynamics under various conditions. These models can predict how changes in one part of a pathway can impact the overall metabolism of the cell.
"Understanding the kinetic principles in biological systems is essential to unravel the complexities of metabolism and develop targeted therapeutics."
Overall, kinetics in biological systems provides critical insights into the functioning of life. Detailed investigations into enzyme kinetics and metabolic pathways enable advancements in medicine, environmental science, and various technological applications.
Computational Modeling in Kinetics
Computational modeling has become an essential part of studying kinetics. It allows researchers to visualize and analyze complex chemical reactions in a way that is often not possible through experimental methods alone. Kinetics equations, which describe the rates of reactions, can have varied behaviors depending on a multitude of factors including concentration, temperature, and the presence of catalysts. Computational tools help in simulating these reactions under different conditions and provide insights into reaction mechanisms.
Modeling offers several benefits:
- Accuracy: Simulations can provide precise predictions of reaction rates and mechanisms, which can lead to more reliable results.
- Efficiency: It is often quicker and less costly to run simulations than to conduct exhaustive laboratory experiments.
- Exploratory Analysis: Researchers can test hypothesized changes such as alterations in concentrations or temperature, without needing to physically run each scenario in the lab.
However, there are considerations to keep in mind. The accuracy of computational models heavily relies on the algorithms used and the quality of the input data. Misinformation or subtle errors can lead to significant deviations from expected outcomes.
Simulating Kinetics Equations
Simulating kinetics equations involves the use of mathematical models to replicate and predict the behavior of chemical reactions over time. This process typically utilizes programs that can numerically solve differential equations representing reaction rates. Commonly used equations include those derived from simple kinetics principles, like first-order or second-order reactions.
A classical approach is the use of ordinary differential equations (ODEs), which represent the change in concentration of reactants and products. Understanding the initial conditions and parameters is crucial, as they will dictate the simulation's output. Here’s a brief example of a first-order reaction:
Where:
- is the concentration of the reactant,
- is the rate constant,
- is time.
This equation can be solved using numerical methods to create a concentration vs time graph, giving insight into the dinamic changes occurring during the reaction.
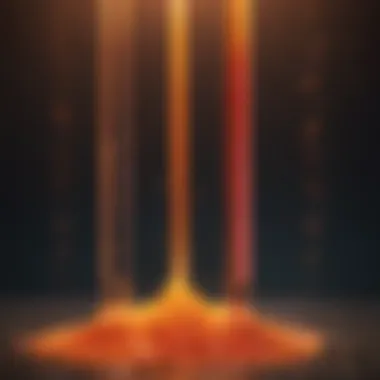
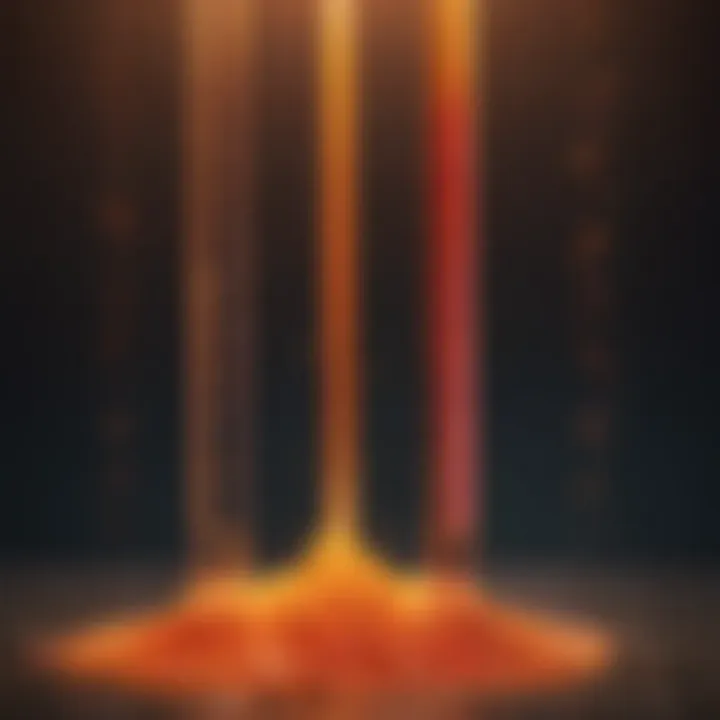
Software Tools for Kinetics Analysis
Many software tools exist for performing kinetic simulations and analysis. Each tool comes with unique features that cater to different aspects of kinetics research. Popular software options include:
- MATLAB: Known for its powerful matrix computation capabilities, MATLAB is widely used for creating custom models for chemical kinetics.
- Chemkin: Specialized for chemical kinetics modeling, it helps in simulating and analyzing reaction mechanisms, particularly in combustion and atmospheric chemistry.
- Phoenix Flow Systems: This software aids in developing kinetic models while providing a user-friendly interface for visualization.
- GROMACS: Primarily used for molecular dynamics, it can be adapted for reactions involved in kinetics studies.
When selecting software, key factors to consider are:
- User friendliness: The learning curve of software should match the user’s expertise.
- Support for complex reactions: Ensure that the chosen tool can handle the specific type of kinetics being studied.
- Community and resources: Active user communities can provide assistance and example cases to better understand and implement various features.
Evaluating Kinetics Data
Evaluating kinetics data is a critical part of understanding how chemical reactions proceed. This section highlights the essential steps that are involved in assessing experimental data related to reaction rates. Proper evaluation allows for greater insight into the mechanisms of reactions, enabling researchers to draw accurate conclusions about the processes at hand. The benefits of evaluating kinetics data are numerous, including improved prediction of reaction outcomes and the ability to adjust conditions for desired results.
In chemical kinetics, it is imperative to adapt a systematic approach when analyzing data. This makes it possible to interpret results accurately and to derive meaningful information regarding the behavior of reactants and products under various conditions.
Data Collection Techniques
Data collection is the first and foremost step when evaluating kinetics data. The choice of technique can significantly influence the quality and reliability of the results obtained. There are several methods researchers can employ:
- Spectroscopy: This involves measuring the concentration of reactants or products over time through techniques like UV-Vis, NMR, or IR spectroscopy. These methods are vital for observing immediate changes in chemical concentrations.
- Chromatography: This technique separates mixtures, allowing for accurate quantification of components at different times during the reaction. Techniques like HPLC or GC can provide valuable data on reaction kinetics.
- Conductometry: Used for ionic reactions, this technique measures electrical conductivity changes as ions are produced or consumed during the reaction, offering real-time feedback on kinetics.
- Volume Measurements: Monitoring gas production or consumption can be effective for reactions that yield gaseous products. This can be done using gas syringes or manometers.
Each of these methods has its own advantages and limitations. It is essential to select an appropriate technique based on the specific reaction and the desired data quality. Consistency and repetition in data collection lead to reliability and robustness in the final analysis.
Analyzing Reaction Mechanisms
Once data collection is complete, the following step is analyzing the reaction mechanisms. This analysis unveils how reactants transform into products, providing insight into the kinetic behavior observed in experiments. Key aspects of this analysis include:
- Rate Laws: Determining the rate law from experimental data is crucial. This involves finding how concentration changes influence reaction rates, which can guide the understanding of the underlying mechanisms.
- Elementary Steps: Investigating elementary steps helps in comprehending how different species interact during the reaction. This breakdown of the overall process allows for a detailed mechanism to be proposed.
- Determination of Activation Energy: By studying the temperature dependence of reaction rates, as described by the Arrhenius equation, one can derive the activation energy, providing insight into the energy barrier for the reaction.
- Identifying Intermediates: Observing unstable intermediates can clarify the reaction pathway. Intermediates can often be detected using specialized techniques, offering valuable data for mechanism elucidation.
Challenges in Kinetics Research
The study of kinetics equations encounters numerous challenges that affect both theoretical understanding and practical applications. Understanding these hurdles is critical for researchers, as they shape the direction of future studies and innovations in the field. Addressing challenges can lead to more accurate modeling of kinetic behavior and enhance methodologies utilized in both laboratory and industrial contexts.
Limitations of Current Models
Current kinetic models often exhibit limitations that may not fully capture the complexities of real-world reactions. One significant challenge lies in the assumptions made during the derivation of these models. For instance, many existing models assume ideal behavior, neglecting factors like reaction reversibility, competing side reactions, and non-ideal conditions. These simplifications can lead to discrepancies between predicted and observed reaction rates.
Moreover, the reliance on empirical data to establish rate laws can introduce biases. If the data used does not represent a broad range of conditions or reactions, the derived models may lack general applicability. Consequently, researchers might struggle with scaling results from lab settings to industrial or environmental applications, which often involve more complex scenarios.
In addition, computational limitations can hinder the analysis of intricate systems with multiple interacting variables. As the number of reactions or species increases, the computational power required grows exponentially, potentially rendering traditional modeling approaches inefficient.
Emerging Research Areas
In light of these challenges, several research areas are emerging that seek to expand our understanding of kinetics. One promising avenue is the incorporation of machine learning into kinetics research, which allows for the analysis of large datasets in ways traditional methods cannot. This data-driven approach could lead to the development of new kinetic models that better reflect complex interactions.
Furthermore, researchers are exploring the role of microenvironments in reaction kinetics. Understanding how factors at the micro-scale, such as particle size and local concentrations, influence reaction rates can refine existing models and improve predictive accuracy.
Another significant focus is the impact of varying reaction conditions, such as pressure and solvent effects, on reaction kinetics. Investigating these aspects can illuminate new pathways for optimizing reactions for industrial applications.
In summary, while the field of kinetics research faces substantial challenges, it also presents exciting opportunities for advancement. By acknowledging limitations and exploring emerging areas, the scientific community can continue to develop a comprehensive understanding of kinetics equations and their applications across diverse fields.
Future Directions in Kinetics Studies
Understanding the future directions in kinetics studies is crucial. As science evolves, so do the methods and applications within this field. Emerging innovations are reshaping how kinetics is studied and applied across various disciplines. This section discusses the promising avenues being explored and the potential impact they may deliver.
Innovations in Kinetics Research
Innovative technologies are transforming kinetics research. The rise of high-throughput screening methods enables researchers to evaluate reaction rates at an unprecedented scale. This acceleration allows for quicker identification of catalysts and optimization of conditions that drive specific reactions. Additionally, advancements in nanotechnology contribute to the development of new materials that enhance catalytic efficiency. For instance, metal-organic frameworks (MOFs) are being utilized for their unique properties in selective catalysis.
Another significant innovation comes from machine learning algorithms. These algorithms can predict reaction outcomes based on historical data, allowing researchers to focus on the most promising pathways. They can analyze vast datasets quickly, bridging gaps in understanding complex reaction dynamics. This application not only increases efficiency but also opens doors to new discoveries in kinetics.
Interdisciplinary Approaches
Interdisciplinary approaches in kinetics studies are noteworthy. Fields such as biology, materials science, and environmental science increasingly influence kinetics research. By collaborating across disciplines, scientists can create comprehensive models that integrate various factors affecting reaction rates.
For example, the melding of enzymology and kinetics has led to better understanding enzyme reactions. This collaboration enhances practical applications like drug development and synthetic biology. Through interdisciplinary research, it is possible to develop more effective and sustainable catalysts that resonate with the growing need for environmentally friendly solutions in chemical production.
Furthermore, integrating computational models with experimental data provides robust frameworks for analyzing kinetics in real-world scenarios. These approaches enable the simulation of complex systems, predicting behavior under varied conditions, which is crucial for both academic research and industrial applications.
"The future of kinetics studies lies in its ability to adapt and evolve through innovations and interdisciplinary collaboration."
The evolution of kinetics studies is vital for our ongoing understanding of chemical reactions. By harnessing new technologies and fostering cooperation among diverse scientific fields, future research can address pressing challenges and contribute meaningfully to advancements in this domain.
Epilogues
In the realm of chemical kinetics, conclusions serve a pivotal role. They synthesize the myriad discussions held throughout the article and distill complex information into digestible insights. By summarizing the key elements explored, conclusions provide clarity on the implications of kinetics equations in scientific research and industrial processes.
A significant aspect of drawing conclusions in this context is recognizing the interconnectedness of various concepts. For instance, the importance of temperature and catalysts cannot be overstated, as they fundamentally influence reaction rates. Understanding these interactions allows for improved applications in fields such as environmental science and industrial chemistry.
The insights derived from kinetics equations are not just academic; they influence practical applications in everyday science.
Moreover, conclusions encourage critical reflection on the failures and limitations of existing models. They highlight areas for future study, guiding researchers toward innovative solutions. This reflection is essential for continual advancement in kinetics research, ensuring relevance in a rapidly evolving scientific landscape.
Benefits of Solid Culminations
- Integration of Knowledge: Conclusions help to tie together various aspects of the subject matter, reinforcing learning and understanding.
- Guidance for Future Research: They pinpoint gaps in current knowledge and suggest new avenues for exploration.
- Practical Implications: Conclusions translate theoretical discussions into applicable knowledge, showcasing the real-world impact of kinetics equations.
Keeping these points in mind is crucial for readers, whether they are students or seasoned professionals. This final section not only wraps up the article but also sets the stage for ongoing exploration in kinetics, embodying the essence of scientific inquiry.
Types of References
References can come in various forms, each serving a unique purpose:
- Journal Articles: These provide peer-reviewed findings specific to kinetics and are often the cornerstone of scientific research.
- Books: Comprehensive texts on chemical kinetics offer a broader academic context and may present theories and equations in greater detail.
- Conference Proceedings: Recent studies often present cutting-edge findings that can be relevant for ongoing research.
- Online Resources: Websites like Wikipedia, Britannica, and relevant forums can provide general summaries and discussions on kinetics but should be approached with discernment regarding their reliability.
"An extensive reference section not only informs but also empowers the audience by providing them tools for independent learning and research."
Considerations for References
When compiling references, it is important to consider:
- Relevance: Ensure sources directly relate to the discussed topics to maintain thematic coherence.
- Recency: In a rapidly evolving field like kinetics, more recent publications tend to provide the latest advances and perspectives.
- Diversity of Sources: A mix of theoretical and practical insights can enrich the discussion.
- Credibility: Prioritize reputable journals and trusted authors to bolster the reliability of the information presented.
In summary, the section on references in this article offers a portal for further exploration into kinetics equations, enhances the legitimacy of the claims made, and serves as a testament to the rich scholarly work that informs our understanding of chemical reaction rates.