Understanding the qPCR Process: Techniques and Uses
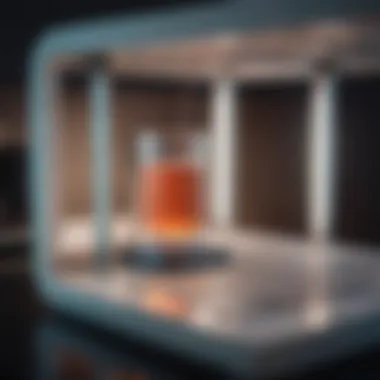
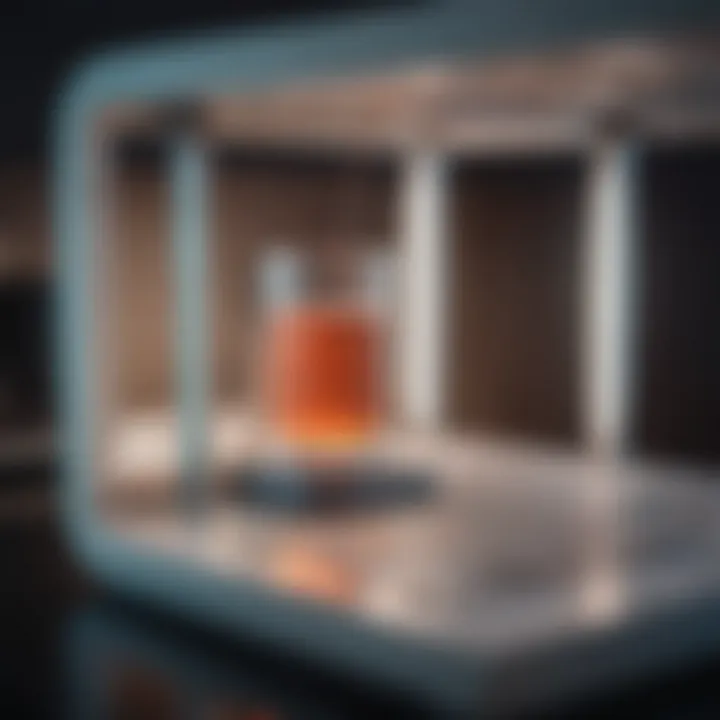
Intro
The world of genetic research has seen a powerful transformation with the advent of quantitative Polymerase Chain Reaction, commonly known as qPCR. This technique, which amplifies DNA with remarkable precision, has unlocked doors to insights in various scientific domains. From evolutionary biology to medicine, it offers tools that can be used to both understand the intricate workings of genetic material and address real-world problems.
In the realm of molecular biology, the importance of qPCR cannot be overstated. As researchers push the boundaries of what we know about genomes, it is crucial to grasp the underlying mechanisms and workflows that make qPCR an indispensable tool. This article provides a detailed exploration of the qPCR process, highlighting methodologies and applications that have come to define modern genetic analysis.
Research Highlights
Key Findings
In the course of exploring qPCR methodologies, a few key findings become evident:
- Efficiency: qPCR significantly enhances the speed and accuracy of DNA quantification compared to traditional methods.
- Versatility: This process is adaptable to a range of applications, including pathogen detection, gene expression analysis, and genotyping.
- Primer Design: The design of primers is a critical factor that influences the success of the amplification process. Properly designed primers can make or break the reliability of results.
Implications and Applications
The implications of qPCR extend far beyond academic circles. Here are some notable applications:
- In clinical diagnostics, qPCR is used to detect diseases early by quantifying viral load or bacterial presence in patient samples.
- Agricultural research relies on qPCR to develop genetically modified organisms with desirable traits.
- The field of forensics employs qPCR to analyze biological samples for identifying individuals or determining relationships among genetic materials.
Methodology Overview
Research Design
A solid understanding of the qPCR process starts with its research design. The typical layout involves a clear definition of the research question followed by the selection of appropriate DNA samples and controls. The design must account for factors like sample type, target genes, and the context in which the qPCR data will be used. This foresight increases the validity and reliability of the findings.
Experimental Procedures
Conducting a qPCR experiment encompasses several essential steps:
- Sample Preparation: Extracting nucleic acids from samples is the first step. This process must be done meticulously to avoid contamination.
- Primer and Probe Setup: Selection and design of specific primers and probes for the target DNA sequences are pivotal. This can involve software tools and databases that aid in ensuring specificity and efficiency.
- Amplification: The qPCR machine is programmed with cycling parameters, which control the temperature changes required for denaturation and extension phases.
- Data Analysis: Post-amplification, the data are analyzed using relative quantification or absolute quantification methods. Software tools facilitate this, allowing researchers to visualize the amplification curves and determine the initial quantity of target sequences.
"The journey to master qPCR is not a sprint; it is a marathon that requires precision, understanding, and patience."
In sum, qPCR stands as a linchpin in genetic research and diagnostics. Its growing list of applications continues to reshape our approach to science, making understanding its principles and methodologies more essential than ever.
Prelims to qPCR
The burgeoning field of molecular biology has pivoted significantly around quantitative Polymerase Chain Reaction, commonly known as qPCR. This powerful tool, harnessed by researchers and diagnostics experts alike, enables precise quantification of DNA and RNA, laying the groundwork for numerous applications across various biological disciplines. Its importance cannot be overstated; qPCR is pivotal in the realms of medical diagnostics, pathogen detection, and genetic research. Through this introduction, we'll unpack the fundamental elements that make qPCR a cornerstone of modern molecular techniques and explore some considerations that reflect its significance in contemporary science.
Definition and Overview
At its core, qPCR is an advanced iteration of the classical Polymerase Chain Reaction (PCR) technology. While traditional PCR primarily focuses on amplifying DNA, qPCR offers real-time measurements of the amplification process. This allows scientists to quantify nucleic acids with remarkable accuracy. To put it plainly, qPCR provides a method not only to make copies of DNA but also to see just how many copies are made during each cycle of the reaction. This sets it apart as a quantifiable technique that has reshaped the landscape of genetic analysis.
The beauty of qPCR hinges on its use of fluorescence. Specific fluorescent dyes or probes bind to the amplified DNA and allow for real-time monitoring of the reaction, effectively turning a qualitative experiment into a quantitative powerhouse. The data gathered can be critical for various applications, from identifying genetic disorders to tracking viral load in infectious diseases.
In summary, qPCR is not just a tool—it's a revolution in how we approach genetic material. With the understanding that it bridges precision and accessibility, the stage is set for its role in the chapters to come.
Historical Development
The journey of qPCR began in the late 1980s with the foundational work of Kary Mullis, who developed PCR itself. Mullis's visionary approach laid the groundwork for expanding molecular biology's capabilities. However, the transition from qualitative PCR to quantitative qPCR unfolded in the mid-1990s. Researchers quickly recognized the limitations of standard PCR for quantification purposes. The potential to accurately measure and monitor DNA in real-time became a focal point for scientific inquiry.
In 1996, the first commercial versions of qPCR became available, largely made possible by advancements in thermal cycler technology and the innovation of fluorescent chemistry. As it gained traction, qPCR found its way into clinical labs and research institutions, signaling a major leap in our ability to study gene expression, genetic mutations, and microbial pathogens.
In subsequent years, qPCR has undergone numerous refinements—making strides in the precision of its measurements and enhancing the ease of usability for researchers. The advent of multiplexing techniques further allowed for the examination of multiple targets in a single reaction, further solidifying qPCR's status as an indispensable tool.
A nod must be given to the evolution leading to the present, where qPCR plays a vital role in landmark discoveries and diagnostics. The historical narrative of qPCR is a testament to perseverance and ingenuity within the scientific community, engaging future generations of researchers to continue this important work.
Fundamental Principles of qPCR
Understanding the fundamental principles of quantitative Polymerase Chain Reaction (qPCR) is essential in grasping its significance in modern molecular biology. At its core, qPCR combines the amplification of DNA with real-time detection, allowing researchers to quantify DNA or RNA more accurately than traditional methods. This section dives into two key components: the mechanism of polymerization and the role of fluorescence.
Mechanism of Polymerization
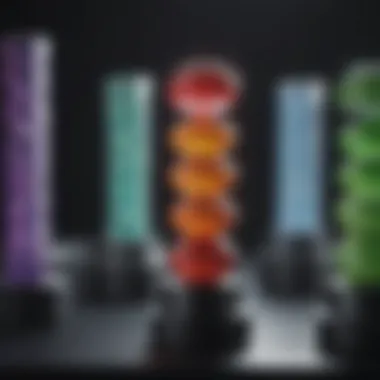
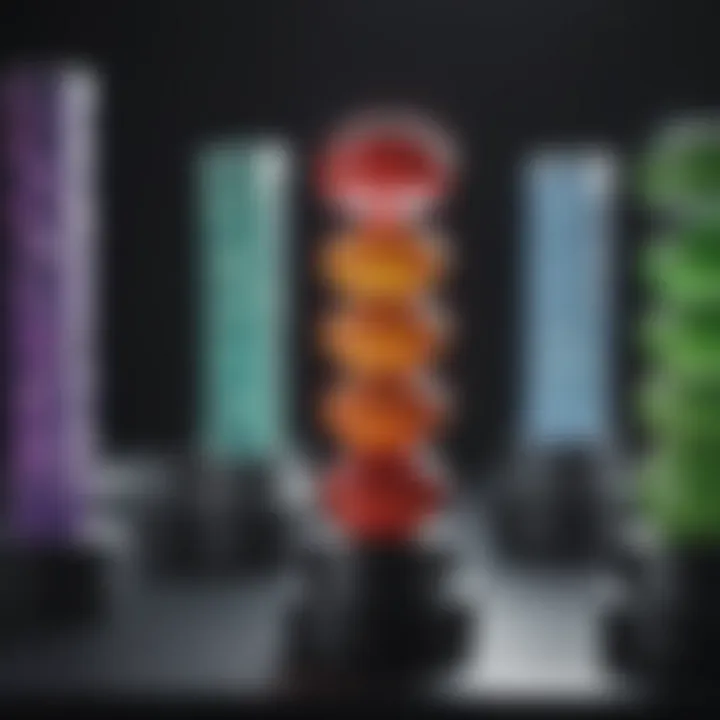
Polymerization in qPCR is the process where DNA or RNA is replicated exponentially. The principle is straightforward yet brilliant: when a sample of nucleic acid is combined with specific primers and a DNA polymerase enzyme, the material undergoes cycles of denaturation, annealing, and extension.
- Denaturation: The double-stranded DNA melts open, separating into single strands. Typically, a temperature of about 94–98°C does the trick.
- Annealing: The temperature is lowered – generally to 50–65°C – allowing primers to attach, or anneal, to their complementary sequences on the single-stranded DNA.
- Extension: Lastly, the temperature is nudged up again, around 72°C, allowing the DNA polymerase to synthesize new strands by extending from the primers. During this process, the amount of DNA increases logarithmically, doubling with each cycle.
This mechanism is crucial as it allows for the rapid and efficient amplification of targeted DNA sequences. Not only is the amplification significant for detection, but it also enhances specificity due to the careful design of primers. Properly crafted primers can make or break an experiment, determining if the process runs smoothly or bogs down due to non-specific binding.
Role of Fluorescence
The role of fluorescence in qPCR cannot be overstated. While amplification generates DNA, fluorescence enables the detection and quantification of the amplified product in real-time. Understanding this interplay is vital in any qPCR analysis.
In a typical qPCR setup, fluorescent dyes or probes are integrated into the PCR mix. These agents emit light when they bind to the amplified DNA. The two primary methods of fluorescence detection are:
- SYBR Green: This dye binds to double-stranded DNA, producing fluorescence upon binding. As more DNA forms, the intensity of the fluorescent signal increases proportional to the amount of DNA being synthesized.
- TaqMan Probes: A more specific approach, TaqMan probes are designed with a reporter dye and a quencher. When the probe is intact, the fluorescence is suppressed. Upon primer extension during the PCR process, the probe is degraded, freeing the reporter dye and generating a measurable signal.
The integration of fluorescence technology into qPCR workflows allows scientists to obtain quantitative data effectively, turning a complex analysis into an easily interpretable graph.
Moreover, fluorescence markers allow for multiplexing, enabling the detection of multiple targets in one reaction. This capacity can save valuable time and resources, making it especially advantageous in clinical diagnostics, where multiple pathogens must be identified simultaneously.
Essential Components of qPCR
In the realm of quantitative Polymerase Chain Reaction (qPCR), three components stand out as absolute necessities: DNA template preparation, primer design and optimization, and the selection of qPCR reagents. Each of these elements serves a vital role in ensuring that the process runs smoothly and efficiently, allowing researchers to gather accurate and reliable data.
DNA Template Preparation
The backbone of any qPCR experiment is the DNA template. It is the substance that carries the genetic material one aims to amplify. Furthermore, the quality and quantity of this template can drastically impact the outcome of the experiment.
To prepare a satisfactory DNA template, one must consider the source of the DNA. Whether it comes from human tissue, bacterial cultures, or environmental samples, the extraction methods will differ. In many cases, researchers employ kits like the Qiagen DNeasy Blood & Tissue Kit to yield high-purity DNA. Additionally, it is critical to assess the concentration and purity of the template using spectrophotometry.
Missteps in this area can lead to non-specific amplifications or, worse yet, failed experiments, making it essential to utilize a clean and enriched DNA sample.
"A well-prepared DNA template is half the battle won in achieving reliable qPCR results."
Primer Design and Optimization
Next up is primer design—think of it as crafting the bolts to fit your screwdriver. Primers are short sequences of nucleotides that initiate the DNA amplification. Their specificity is key; poorly designed primers can lead to non-specific bindings, which muzzle the accuracy of quantification.
When designing primers, several factors must be taken into account:
- Length: Typically, primers are around 18-24 nucleotides long.
- Melting Temperature (Tm): Tm should be similar for both forward and reverse primers to ensure they bond uniformly during the amplification.
- GC Content: Ideally between 40-60%, providing stability and flexibility.
Optimization is just as crucial. Running a gradient PCR can help ascertain the ideal annealing temperature, balancing specificity with yield.
Selection of qPCR Reagents
Finally, the choice of qPCR reagents is pivotal for the experiment's success. The main components include DNA polymerase, dNTPs, buffer solutions, and fluorescent dyes, among others. Each plays a distinct role in the process:
- DNA Polymerase: High-fidelity enzymes like Taq polymerase are preferred for their ability to withstand high temperatures while maintaining accuracy.
- dNTPs: These are the building blocks for new DNA strands; quality can affect the overall efficiency of the reaction.
- Fluorescent Dyes: SYBR Green or TaqMan probes enhance the detection of amplified DNA, allowing for real-time monitoring of the qPCR process.
Choosing the right reagents simplifies protocols and enhances the probability of obtaining reliable data. Ignoring this step can lead to poor results or missing out on capturing nuanced biological variations.
In summary, the essential components of qPCR hinge on meticulous preparation and selection. As researchers tread the path of quantifying genetic material, understanding these foundational elements can make all the difference, turning steps into strides.
The qPCR Workflow
The qPCR workflow represents the backbone of quantitative Polymerase Chain Reaction, intertwining various meticulous steps that ensure reliable amplification and quantification of DNA. The efficiency and accuracy of the entire process hinge on three crucial elements: sample preparation, thermal cycling protocols and data acquisition and analysis. Understanding these dimensions is pivotal for researchers and practitioners, as they are integral to achieving reproducible and interpretable results in various applications ranging from medical diagnostics to genetic research.
Sample Preparation
Sample preparation is the first crucial step in the qPCR workflow. It involves isolating and purifying nucleic acids from biological samples, which may include blood, tissues, or even environmental samples. The quality and integrity of the DNA template can significantly impact the subsequent amplification process. Here are some essential points to consider:
- Quality Matters: Using high-quality templates is vital. If the DNA is degraded or contaminated, it could lead to poor amplification yields and skewed results. Therefore, methods like phenol-chloroform extraction or silica column purification are often employed to ensure purity.
- Concentration Assessment: After extraction, it's important to quantify the nucleic acids using spectrophotometry or fluorometry, so researchers know how much template to use in their reactions. Too much template can inhibit the reaction, while too little can lead to suboptimal amplification.
- Preparation for Reactions: Following quantification, samples are diluted to the appropriate concentration for qPCR, typically between 1 and 100 ng/µL, depending on the assay design.
Thermal Cycling Protocols
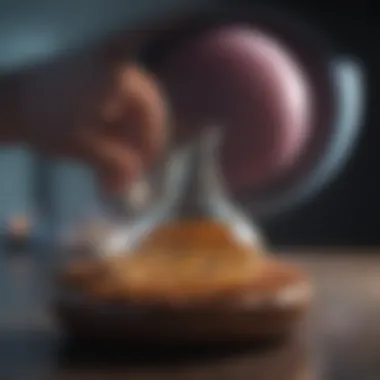
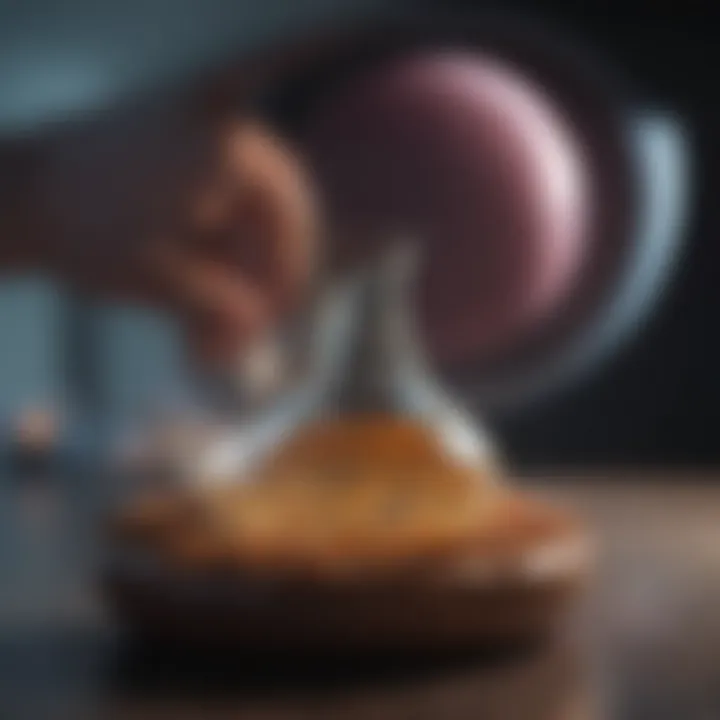
Once the samples are prepped and ready to roll, the next phase is thermal cycling. This is where the magic happens, as DNA is copied exponentially, allowing even small amounts of starting material to be amplified to detectable levels. Thermal cycling protocols include specific temperature and time settings that are crucial for denaturation, annealing, and extension of the DNA strands. Here are the essential phases:
- Denaturation: Usually set at around 94-98°C, this step separates the DNA double strands. It's akin to unzipping a zipper to access the essential data within.
- Annealing: During this phase, temperatures are lowered to about 50-65°C, allowing primers to bind the specific regions of interest on the single-stranded DNA. The choice of temperature impacts the specificity and efficiency of primer binding.
- Extension: Lastly, the temperature is raised to roughly 72°C, facilitating the enzyme Taq polymerase to elongate the new strand by adding nucleotides. The length and accuracy of this phase directly influence the final DNA yield.
A successful qPCR run typically consists of 30 to 40 cycles, enabling a logarithmic increase in the target DNA, which can then be quantified.
Data Acquisition and Analysis
The final stage of the qPCR workflow encompasses data acquisition and analysis, which is as sophisticated and nuanced as the preceding steps. After amplification, the data generated helps deduce the quantity of target DNA in the original sample, alongside the necessary statistical interpretations necessary for validation. That process includes:
- Fluorescence Monitoring: During amplification, fluorescence signals are captured in real time. The intensity of these signals correlates to the amount of DNA produced, which is crucial for quantification.
- Threshold Cycle (Ct) Determination: The point at which fluorescence surpasses a predetermined threshold indicates the Ct value. This number is utilized to infer the initial quantity of target molecules in the sample.
- Data Interpretation: Statistical tools such as standard curves and comparative Ct methods (ΔΔCt) help outline how much of the target was present in the original sample. Ensuring statistical rigor in this step is vital for reliable and reproducible results.
In summary, understanding the intricacies of the qPCR workflow—from sample preparation through to data analysis—is essential for producing quality results that stand up to scientific scrutiny. These stages are not merely administrative; they are critical touchpoints that collectively determine the success of the qPCR experiment, influencing everything from diagnostic accuracy to advances in genetic research.
Quantification Methods in qPCR
Quantification methods in quantitative Polymerase Chain Reaction (qPCR) represent the backbone of this powerful tool for measuring nucleic acids. Understanding these methods is crucial for students, researchers, and professionals concerned with accurate data analysis in various fields, such as molecular biology, diagnostics, and genetic research. The methods can help ascertain not just the presence of a specific DNA product but also its exact quantification. Such detailed insight can significantly impact research outcomes, influencing everything from basic scientific understanding to clinical decision-making.
Absolute vs. Relative Quantification
The distinction between absolute and relative quantification is fundamental for anyone delving into qPCR applications. Absolute quantification refers to the precise measurement of the amount of a target nucleic acid in a sample, typically expressed in copies per microliter. To achieve this, standard curves generated from known quantities of DNA serve as a reference. For instance, researchers can create a standard curve by preparing a series of dilutions of a template DNA, allowing them to correlate fluorescence intensity with specific DNA concentrations.
On the other hand, relative quantification focuses on comparing the expression of a target gene against a reference gene, often known as housekeeping genes. It does not provide exact quantities but quantifies the difference in expression levels, making it simpler for determining gene regulation. This method is often used in studies where comparing expression levels across different conditions is paramount.
Standard Curves
Standard curves are a lifeline for achieving accuracy in absolute quantification. The creation of a standard curve not only validates the accuracy of the results but also ensures reproducibility across experiments. Generating a standard curve involves creating a series of known concentrations of nucleic acid, plotting them to obtain a linear relationship with the cycle threshold (Ct) values from your qPCR run.
In practice, each standard curve should ideally mirror the target sample's conditions to yield reliable results. If a standard curve is not aligned with the test conditions, the quantification may lead to figures far from the truth. Factors impacting the effectiveness of these curves include the quality of reagents, PCR efficiency, and thermal cycling conditions.
"Standard curves are not just a formality, they're your best friends in the world of qPCR quantification."
Quality Control Assessments
Quality control assessments are non-negotiable in ensuring the integrity and reliability of qPCR results. These assessments touch on various aspects of the qPCR process, including verifying the correct design of primers and confirming the absence of inhibitors that can skew results.
Several techniques are utilized for quality control. One common practice is the inclusion of no-template controls (NTC), which helps to identify any contamination in the reagents being used. Moreover, the efficiency of the qPCR reaction can be monitored through replication processes, ensuring consistent and reliable Ct values. Additionally, integrating multiple reference genes can help normalize the data, especially in relative quantification contexts, thereby mitigating variability.
By including stringent quality control measures, researchers can trust that their findings are reflective of the true biological state they are investigating, allowing for more robust conclusions and applications in further studies.
In sum, mastering the quantification methods in qPCR is essential for any scientific inquiry involving nucleic acid measurement.
Applications of qPCR
Quantitative Polymerase Chain Reaction (qPCR) stands as a cornerstone in various scientific fields, playing a pivotal role in enhancing our understanding of biological mechanisms and diseases. This potent technique allows researchers to quantify DNA or RNA in a sample with high specificity and sensitivity. The implications of qPCR stretch from clinical diagnostics to the realm of genetic research, each application showcasing the method’s versatility. Below, we will delve into specific applications of qPCR, highlighting their significance, benefits, and the considerations they entail.
Medical Diagnostics
In the world of medical diagnostics, qPCR shines as an invaluable tool. Its capacity to detect and quantify gene expression levels enables clinicians to diagnose conditions such as cancer, genetic disorders, and infectious diseases with remarkable accuracy. For instance, when physicians suspect a viral infection, a qPCR test can quickly ascertain viral load, guiding treatment decisions effectively.
The speed of qPCR results is another compelling benefit. Unlike traditional diagnostic methods, which may take days to provide results, qPCR can often deliver findings within hours. This rapid turnaround is crucial in acute settings, such as determining the best course of treatment for a patient during a severe illness. However, it is essential to be mindful of the potential for false positives or negatives, emphasizing the necessity for proper controls and validation within the tests.
"The precision of qPCR not only improves diagnostic accuracy but can also influence patient outcomes significantly."
Pathogen Detection
Pathogen detection is another realm where qPCR reigns supreme. In both clinical and environmental settings, the ability to detect minute quantities of nucleic acids from pathogens can be life-saving. With qPCR, laboratories can identify bacterial and viral pathogens responsible for outbreaks in a timely manner, helping to contain and manage public health crises.
Furthermore, qPCR doesn't only apply to human pathogens; its utility extends to agricultural contexts too. Detecting plant pathogens can prevent devastating crop losses, ultimately protecting food supply chains and farmers’ livelihoods. Various pathogens, such as Phytophthora and Xanthomonas, are now routinely screened using qPCR methods. Still, one must consider contamination factors and develop robust sampling techniques to ensure accurate results.
Genetic Research
In the sphere of genetic research, qPCR has transformed the way scientists explore gene expression and regulation. By allowing researchers to analyze the quantity of specific mRNAs, qPCR offers insight into how genes respond to environmental changes or treatments. This capability is particularly valuable in pharmacogenomics, enabling personalized medicine approaches tailored to individual genetic profiles.
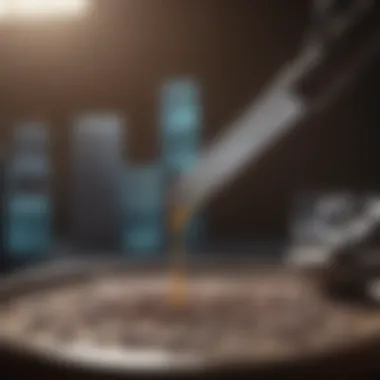
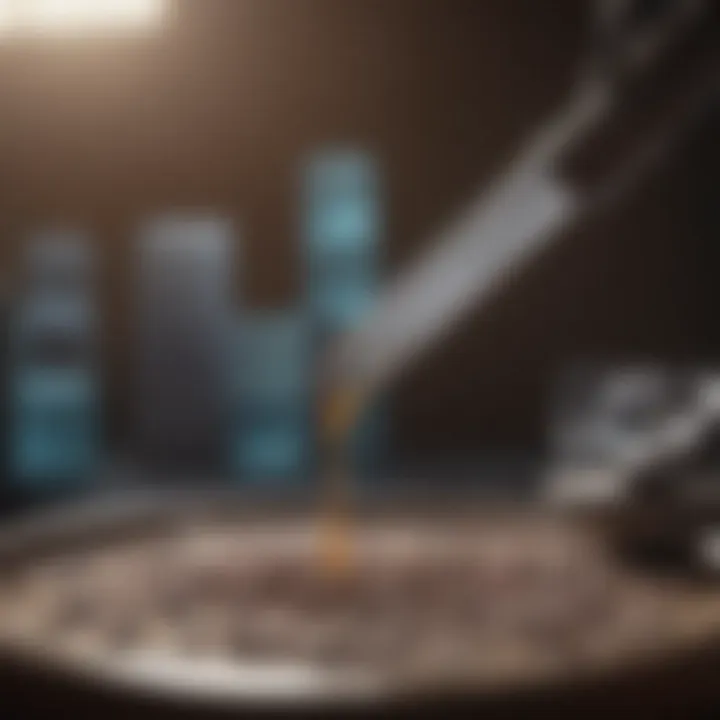
Moreover, advancements in qPCR methodologies, such as multiplex qPCR, allow simultaneous quantification of multiple targets within a single reaction, which can streamline research processes significantly. While qPCR brings with it great benefits, researchers need to remain cautious regarding sample integrity, proper primer design, and validation of results to avoid skewed interpretations of their findings.
Challenges in qPCR
In the realm of qPCR, the challenges faced can often be as significant as the methodologies themselves. Navigating through these obstacles is crucial for achieving reliable and consistent results. Understanding the common issues helps researchers and practitioners mitigate risks and refine their techniques. This section delves into two of the predominant challenges: contamination and inhibitory substances.
Contamination Issues
Contamination can derail even the most precisely designed qPCR experiments. It's often the silent enemy lurking in the corners of the lab. When foreign DNA contaminates samples or reagents, it can lead to false positives or unexpected amplification results. Many labs employ rigorous protocols to reduce contamination, such as using separate areas for pre- and post-PCR reactions.
- Sources of Contamination:
- Environmental factors, including airborne particles.
- Contaminated reagents or equipment.
- Operator handling mistakes, like improperly sealed tubes.
Addressing these aspects is essential to maintain the integrity of experiments. Common strategies used include wearing gloves, using dedicated pipettes for PCR, and implementing regular cleaning procedures with bleach or other disinfectants. Each of these measures serves to protect samples and create a more reliable environment.
"In many qPCR experiments, contamination is the thin line between success and frustrating failure."
Inhibitory Substances
Inhibitory substances present another hurdle that can impact the accuracy of qPCR results. These are elements present in the sample that interfere with the polymerase activity, leading to under- or overestimations of target DNA concentrations. This is particularly common in samples extracted from biological materials.
- Common Inhibitors:
- Hemoglobin from blood samples
- Polyphenols in plant extracts
- High concentrations of salts
To combat the effects of these inhibitors, researchers may need to optimize their DNA extraction protocols or use commercial kits designed to enhance removal of such substances. It involves a fine balance, as over-extraction or purification may result in loss of target material. Adjusting the reaction conditions, such as increasing enzyme concentrations or adding enhancer reagents, can sometimes lead to improved results.
Future Perspectives of qPCR
The landscape of quantitative Polymerase Chain Reaction (qPCR) is evolving rapidly, and it is crucial to explore its future perspectives. As we unlock the nuances of molecular biology, the need for sophisticated and reliable techniques becomes crystal clear. Innovations in qPCR methodologies not only enhance its current applications but also pave the path for new realms of discovery in both research and clinical settings.
Innovative Technological Advances
One cannot ignore the role of technological strides in shaping the future of qPCR. Recent advances are all about enhancing sensitivity and specificity while reducing the time and costs. For instance, digital PCR (dPCR) is gaining traction within the scientific community. This technique allows absolute quantification of nucleic acid without the need for standard curves. It provides a game-changing approach where even rare mutations can be quantitatively analyzed, thereby expanding the utility of qPCR in precision medicine.
Moreover, improvements in thermocyclers—favoring real-time feedback—equip researchers with the tools to monitor reactions on-the-fly. These devices often incorporate advanced features like automated data collection and analysis. This can be especially beneficial when handling multiple samples simultaneously. Altogether, such innovations make qPCR an even more competitive tool in diagnostic laboratories.
Integration with Other Biotechnologies
As diverse bioscience techniques become intertwined, the integration of qPCR with other biotechnologies signifies a fruitful direction for future development. Merging qPCR with next-generation sequencing (NGS) is one such approach that can revolutionize diagnostics. The combination lets researchers broadly assess the genetic landscape of organisms, identifying not just the presence of target sequences but also variations and mutations.
Furthermore, qPCR can augment CRISPR-based methodologies. With CRISPR’s gene-editing prowess, coupling these techniques allows scientists to investigate gene expression quantitatively post-editing, providing essential insights for developing therapies.
The convergence of qPCR with bioinformatics tools also showcases potential. By harnessing data analysis software, researchers can interpret vast datasets more efficiently, enhancing the precision of their findings. These integrations mark a promising frontier, enabling a more holistic understanding of molecular dynamics and genetic interplay in various contexts.
"As qPCR technology continues to advance, its applications will expand, enabling deeper insights into genetic Information that could shape the future of medicine and research."
End
In wrapping up our exploration of the qPCR process, it’s essential to recognize the significance of the findings detailed in this article. The insights gained are not just academic; they hold substantial implications for a variety of fields, ranging from medical diagnostics to environmental monitoring. Understanding the intricate methodologies and applications of qPCR allows researchers to deploy this powerful tool effectively in their work.
qPCR is not merely a laboratory technique; it serves as the backbone for innovations in genetic research and diagnostics. Its ability to precisely quantify DNA and RNA has sharpened our understanding of numerous biological processes, underscored by the meticulous attention given to each critical step—from the careful design of primers to the dynamic analysis of results. As research moves forward, keeping an eye on potential developments in this area will be vital.
The challenges associated with qPCR, such as contamination and the presence of inhibitory substances, cannot be overstated. Yet, through continuous refinement of protocols and the application of novel technologies, researchers can overcome these hurdles, ensuring more reliable results.
In short, the conclusion drawn from the examination of qPCR leads us to a world that is rife with possibilities. The methodologies developed through this process not only equip practitioners with the skills they need but also inspire ongoing conversations about the future direction of genetic research.
Summary of Key Points
- qPCR stands as a crucial method in molecular biology, demonstrating its flexibility and precision.
- Key components include meticulous sample preparation, primer optimization, and selection of reagents—all of which feed into the overall effectiveness of the method.
- The data analysis phase is pivotal, offering insights that can drive critical decisions in clinical and research settings.
- Despite challenges like contamination, ongoing advancements offer hope for greater reliability and innovation in applications of the technology.
"The ability to quantify genetic material with accuracy sets qPCR apart as a transformative tool in biological sciences."
Implications for Future Research
The horizon of qPCR is expanding, revealing new pathways for exploration and innovation. As we glance into the future, several trends appear poised to shape the landscape:
- Integration with Next-Generation Sequencing: This merging could enhance specificity, allowing researchers to obtain comprehensive genetic profiles from minimal sample amounts.
- Adaptation of Digital qPCR Technologies: These techniques promise finer quantification of nucleic acids, providing opportunities for extremely sensitive assays.
- Application in Environmental and Field Studies: Going beyond traditional lab settings, researchers might harness qPCR for real-time monitoring of pathogen outbreaks or agricultural health, thus bridging laboratory science with practical field applications.
- Biosensor Developments: Technological advancements could spur the creation of biosensors utilizing qPCR principles, offering rapid and on-the-spot analysis in various fields including healthcare and food safety.
In summary, the future of qPCR is not just about refining existing methods but also reevaluating and expanding its potential applications. As researchers delve deeper into these implications, the role of qPCR in advancing scientific inquiry will undoubtedly evolve, establishing new benchmarks and opportunities in the realm of genetics.